ABSTRACT
Objective
Acoustic droplet vaporization (ADV) is the liquid-to-gas phase transition of perfluorocarbon (PFC) droplets to microbubbles upon ultrasound insonation. After ADV, gases dissolved in the surrounding fluid diffuse into microbubbles, enabling oxygen scavenging. Characterization of oxygen scavenging and transition efficiency (TE) in whole blood has so far been limited. In this work, oxygen scavenging and perfluorocarbon droplet TE in a saline buffer and whole bovine blood were evaluated using blood-gas analysis and flow cytometry.
Methods
Oxygen scavenging from whole blood via ADV was determined using an in vitro flow phantom with droplets comprising a phospholipid shell and either a decafluorobutane (DFB) or a perfluoropentane (PFP) core. Fluorescent droplets were used to determine ADV TE in whole blood via flow cytometry. Finally, a mathematical model predicting oxygen scavenging from whole blood was developed based on the experimental TE values.
Results
DFB droplets enabled greater oxygen scavenging and higher TE when compared with perfluoropentane droplets in both buffer and whole blood. Increasing the droplet concentration resulted in a greater amount of hemoglobin-bound and dissolved oxygen scavenging from whole blood. ADV of DFB droplets at a concentration of 5 × 10 −4 mL/mL yielded a total oxygen reduction of 913 μM. The TE decreased with increasing droplet concentration in both buffer and whole blood. Experimental oxygen scavenging data in whole blood aligned with the predicted values from the mathematical model.
Conclusion
Increased oxygen scavenging and TE were achieved with DFB droplets relative to perfluoropentane droplets.
Introduction
Acoustic droplet vaporization (ADV) is the phase transition of liquid perfluorocarbon droplets into gas microbubbles in a controlled manner upon exposure to ultrasound [ ]. After ADV, the newly created gas microbubbles scavenge gases, including oxygen, from the surrounding fluid [ ]. ADV-mediated oxygen scavenging technology has been hypothesized to prevent myocardial reperfusion injury by enabling controlled hypoxic reperfusion [ ]. Reperfusion injury is, in part, caused by the formation of the reactive oxygen species after the restoration of blood flow to the myocardium. Therefore, temporarily reducing the amount of bioavailable oxygen during reperfusion (i.e., employing controlled hypoxic reperfusion) has potential cardioprotective effects [ , ].
To enable future cardioprotection studies for assessing the effectiveness of controlled hypoxic reperfusion using ADV, rigorous measurements of the change in oxygen in blood needs to be used. In arterial whole blood, approximately 98% of all O 2 is bound to hemoglobin inside red blood cells, and only approximately 2% of O 2 is dissolved in the plasma [ ]. Prior studies relied on hemoglobin-oxygen saturation (sO 2 ) calculations from partial pressure of oxygen (pO 2 ) measurements [ ]. However, the affinity of hemoglobin to oxygen is altered by blood pH, temperature, partial pressure of carbon dioxide (pCO 2 ) and other factors [ ]. Therefore, direct and simultaneous measurements of pO 2 and sO 2 are needed to understand ADV-mediated oxygen scavenging in physiologic whole blood.
ADV transition efficiency (TE) is defined as the fraction of droplets that have phase transitioned into microbubbles after ultrasound exposure relative to the droplet concentration before ultrasound exposure [ , ]. The TE can be calculated based on number-weighted or volume-weighted changes in droplet concentration [ , ]. The ADV TE is related to, but distinct from, the ADV pressure amplitude threshold [ ]. ADV TE has been shown to depend on the probability of droplet vaporization given particular droplet and ultrasound parameters [ , ]. For instance, Lo et al. [ ] demonstrated that the occurrence of ADV increases with increasing pressure amplitudes. Fabiilli et al. [ ] showed that increasing the number of passes of droplets circulated through an ultrasound field resulted in an increased fraction of vaporized droplets. Additionally, several groups have shown that, as the boiling point of the perfluorocarbon used increases, the TE or the ADV pressure threshold decreases [ ]. For instance, Aliabouzar et al. [ ] showed that nucleation of perfluoropentane (PFP) droplets required a lower ultrasound pressure to trigger vaporization and that drug release from lower boiling point droplets was more efficient. Wu et al. [ ] found that more octafluoropropane droplets phase transitioned into microbubbles than decafluorobutane (DFB) ones did. Benton et al. [ ] found that PFP droplet TE increases with increasing droplet diameter. However, phase-transitioned microbubbles from sub-micron droplets are less likely to cause gas embolization in vivo because their diameter would be similar to that of an erythrocyte [ ]. Thus, comparing the TE of sub-micron droplets consisting of perfluorocarbons (PFCs) with two different boiling points can yield insight into the therapeutic feasibility and efficiency of ADV.
The ADV pressure amplitude threshold has been measured in whole blood [ ]. However, the ADV TE has not been measured in blood, owing to the overwhelming number of particles (e.g., lipid particles and antibody aggregates) and cellular components of blood (e.g., erythrocytes and platelets) in the range of 0.1 to 10 µm. Hemocytometers [ ], Coulter counters [ , ] and dynamic light scattering devices [ , ] have been used conventionally to determine droplet concentration and size distribution in a sample, but these sizing methods cannot differentiate between droplets and the cellular components in blood easily. Indirect measurements of ADV TE, such as B-mode grayscale amplitude or drug release from double emulsions have been used, but only provide qualitative estimates of the TE [ , ]. A direct measurement of ADV TE in blood is lacking.
Motivated by these gaps in knowledge, the ADV-mediated oxygen scavenging and TE in both Krebs-Henseleit buffer (KHB) and physiologic whole blood was measured in vitro . Fluorescent and non-fluorescent lipid-shelled droplets were manufactured with either PFP or DFB cores via high-shear homogenization to facilitate differentiation of droplets from formed elements in blood using flow cytometry. Oxygen scavenging was assessed in KHB and whole blood using dissolved oxygen sensors and a blood gas (BG) analyzer, respectively. Droplet TE was assessed in KHB using a Coulter counter. Finally, a model to predict oxygen scavenging in whole blood based on initial pH, temperature and TE was developed and compared with experimental measurements. Several key hypotheses guided the experimental design in this study. First, we hypothesized that an increase in oxygen scavenging and a decrease in TE would be observed with increasing droplet concentration based on published findings [ ]. Second, droplets containing a lower boiling point PFC (DFB) were hypothesized to exhibit a higher TE than PFP droplets based on prior studies demonstrating increased TE with decreasing PFC boiling points [ , , ]. Last, we extended the oxygen scavenging model for buffers developed by Radhakrishnan et al. [ ] to include the hemoglobin–sO 2 model of Clerbaux et al. [ ].
Methods
Bovine blood and buffer preparation
Differences in the O 2 affinity of hemoglobin between human and bovine whole blood exist; however, these differences were similar to variability observed in humans [ ]. Therefore, donor bovine whole blood was used in this study owing to its cost effectiveness and local availability relative to human blood. Bovine whole blood anticoagulated in citrate phosphate dextrose (30%) was obtained from Lampire Biological Laboratories (Pipersville, PA, USA). The donor blood was shipped on ice the same day it was obtained and received the next morning. Upon arrival, the blood was aliquoted into 50 mL conical vials and stored in a 4°C refrigerator for up to 8 d [ , ].
Before each experiment, 100 mL (sO 2 curve generation) or 150 mL (ADV experiments) aliquots of blood were warmed to 37°C in an uncovered glass beaker and stirred with a magnetic stir bar (250 rpm) for 20 min to equilibrate the blood to average human body temperature and gas saturation at 1 atm. Blood (approximately 2 mL) was drawn with a sterile 3 mL plastic syringe and the pH, pO 2 , hemoglobin sO 2 , pCO 2 and hemoglobin concentration were measured with a BG analyzer (Nova StatPrime Plus analyzer, PP141918OC, Nova Biomedical, Waltham, MA, USA). If the pH reading was below 7.1, 1.5 mL of NaHCO 3 − (1 mL/eq; 84 mg/kg) was added, and another measurement was taken. Addition of 0.5 mL of NaHCO 3 − was repeated until the pH reached at least 7.1. The blood was subsequently passed through a membrane oxygenator, and when 7.1 <SPAN role=presentation tabIndex=0 id=MathJax-Element-1-Frame class=MathJax style="POSITION: relative" data-mathml='≤’>≤≤
≤
pH <SPAN role=presentation tabIndex=0 id=MathJax-Element-2-Frame class=MathJax style="POSITION: relative" data-mathml='≤’>≤≤
≤
7.2, 2% CO 2 was infused into the membrane oxygenator. If 7.2 < pH <SPAN role=presentation tabIndex=0 id=MathJax-Element-3-Frame class=MathJax style="POSITION: relative" data-mathml='≤’>≤≤
≤
7.45, 3%–4% CO 2 was infused. If the pH was >7.45, 6%–8% of CO 2 was infused. These values were selected based on pilot experiments, which demonstrated that under these conditions, the blood would have a pH between 7.35 and 7.45 upon exiting the membrane oxygenator. This is the standard pH range for both human and bovine arterial blood [ , , ].
KHB (10× concentration) was prepared with 119 mmol/L NaCl, 4.7 mmol/L KCl, 1.2 mmol/L MgSO 4 , 1.25 mol/L CaCl 2 and 1.2 mmol/L KH 2 PO 4 . On the day of the experiment, a 1× KHB solution was prepared by diluting 10× KHB 1:10 with deionized water and adding NaHCO 3 − and glucose to final concentrations of 25 mmol/L and 11 mmol/L, respectively. The KHB was warmed in a water bath to 37°C before the start of each experiment.
In vitro flow phantom
An in vitro flow phantom was composed of water jacketed tubing as in a flow phantom setup described in Benton et al. [ ] ( Fig. 1 ). The membrane oxygenator was composed of 12 m of gas-permeable silicone tubing (1.47 mm inner diameter, 0.23 mm wall thickness Reference #: 60-011-06, HelixMark, Carpinteria, CA, USA) ( Fig. 1 ). Oxygen (O 2 ), carbon dioxide (CO 2 ) and nitrogen (N 2 ) set to 0%–15%, 0%–8% and 70%–100%, respectively, were infused into the membrane oxygenator 15 min before each experiment to allow the oxygenator to equilibrate. These ranges had been determined in pilot experiments. The total flow rate of gas infusion was 500 mL/min, and the gases were mixed with a gas blender (model GB-103, MCQ Instruments, Rome, Italy). Low N 2 and high O 2 (e.g. 70% N 2 and 15% O 2 ) were infused when a low pO 2 and low sO 2 was being achieved, and vice versa. Whole blood was maintained at 36.5–37.5°C and circulated through the flow phantom containing the membrane oxygenator at 13.4 mL/min ( Fig. 1 ). Temperature was measured with a thermocouple (5SRTC, Omega Engineering, Stamford, CT, USA). The BG analyzer was used to measure the pO 2 and sO 2 .
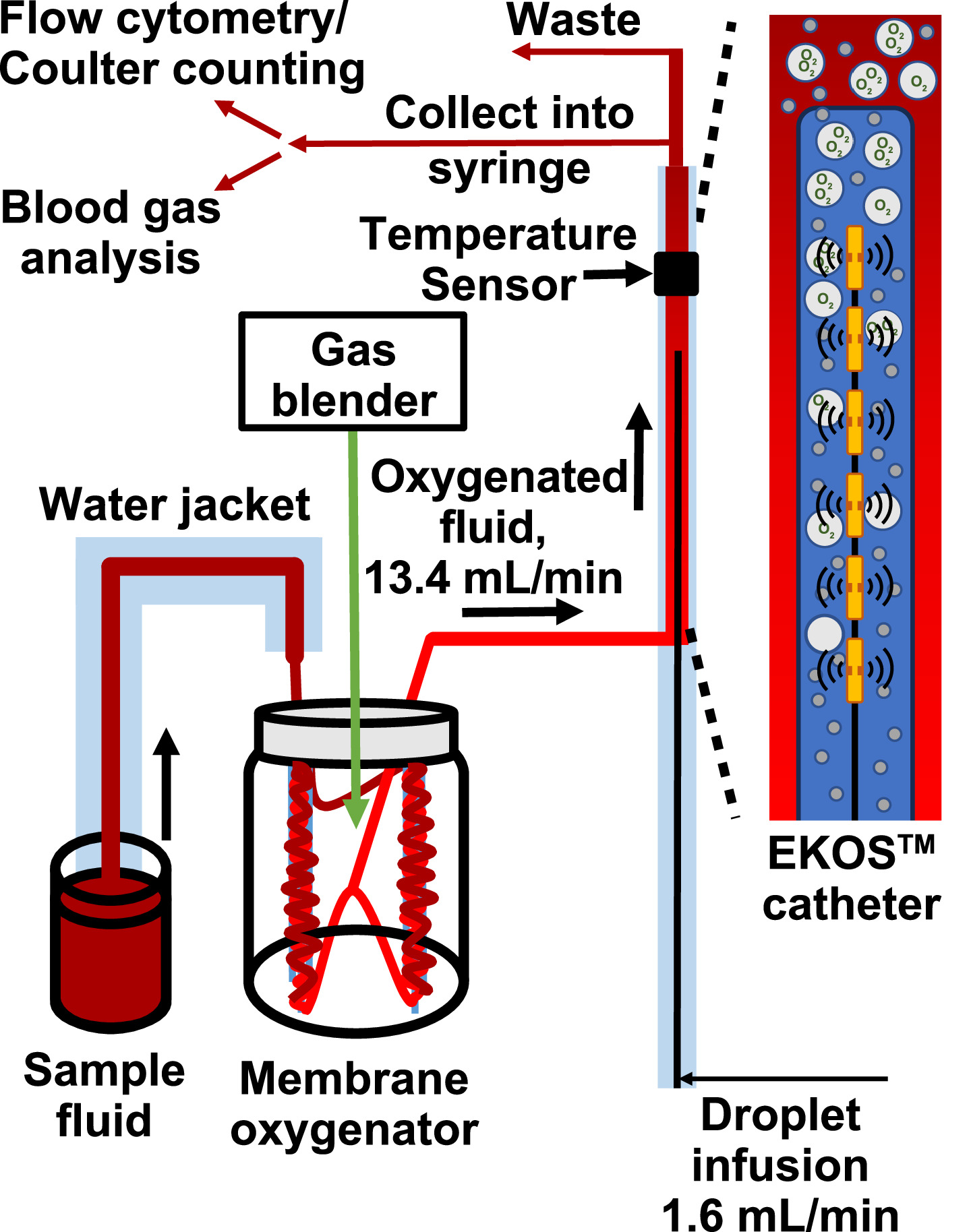
Phospholipid perfluorocarbon droplets manufacturing and sizing
Lipid PFP or DFB (FluoroMed, L.P. Round Rock, TX, USA) droplets were manufactured using high-shear homogenization with phospholipids [ ]. Phospholipids were purchased from Avanti Polar Lipids (Alabaster, AL, USA) and BroadPharm (San Diego, CA, USA). We dissolved 1,2-disterayol-sn-glycero-3-phosphocoline (DSPC), 1,2-distearoyl-sn-glycero-3-phosphoethanoamine-N-[methoxy (polyethyleneglycol)-2000] (DSPE-PEG2000) and DSPE-PEG2000-Fluor 647 in chloroform (25 mg/mL). Non-fluorescent droplets were made with DSPC and DSPE-PEG2000 at a molar ratio of 9:1 and a final concentration of 2.5 mg/mL total lipids. Fluorescent droplets were made with DSPC, DSPE-PEG2000 and DSPE-PEG2000-Fluor 647 with a molar ratio of 9:0.96:0.04, respectively. A thin lipid film was formed in a round-bottom flask submerged in a water bath heated to 41°C using a rotary evaporator (N1001-S, EYELA, Bohemia, NY, USA) with gentle nitrogen stream for 1–2 h. Filtered 1× phosphate-buffered saline (PBS), propylene glycol and glycerol in a 16:3:1 v/v/v ratio were added to the flask with the thin lipid film. To enable a complete dissolution of the phospholipids, the solution was subsequently heated in a 70°C water bath for 15 min without sonication and 15 min with sonication, (FisherBrand 11203, Fisher Scientific, Rockford, IL, USA) using a sweep mode setting at 37 kHz and a 100 W power setting. The excipient solution was cooled for 10 min at 4°C, and 2 mL was aliquoted into a 2 mL glass serum vial pre-cooled to 4°C. The vials were capped with a rubber septum and crimped. DFB was condensed via flow at 20 psi into 10 mL crimped serum vials covered in dry ice. Afterward, 400 µL of condensed DFB or 250 µL of PFP were added using a 1 mL syringe. These PFC volumes were determined in pilot experiments to optimize our droplet manufacturing protocol. Using 400 µL of PFP resulted in considerably larger droplets (1.3–1.4 µm) than those made with 400 µL of DFB (0.9–1.0 μm). Therefore, 250 µL of PFP, which was found to produce a similar droplet size distribution as 400 µL of DFB, was used. The mixture was cooled on ice for at least 10 min. Overnight mixture storage was in a 4°C refrigerator. A coarse emulsion was formed by high-speed shaking each vial for 45 s (VIALMIX, Lantheus Medical Imaging, Billerica, MA, USA) and then cooled on ice for at least 20 min. The coarse emulsion was cooled in a dry ice/isopropanol bath at −20°C until the solution could be pipetted into a syringe without dripping from a pipette tip. Lipid droplets were formed by passing the coarse emulsion once through a direct high-pressure homogenizer pre-cooled to 4°C (LV-1 Microfluidizer, Microfluidics, Westwood, MA, USA) at 13,000 psi [ , ].
To obtain PFP droplets with a similar size distribution to the DFB droplets, 250 µL of PFP was added to 2 mL of lipids in the serum vial. No dry ice bath coarse emulsion stabilization was necessary before high-pressure homogenization because PFP did not evaporate at 4°C. The solution was shaken to obtain a coarse emulsion, cooled on ice for 20 min and passed once through the high-pressure homogenizer at 13,000 psi.
Droplet size distributions and volume-weighted concentrations were determined with a Coulter counter (Multisizer 4 or Multisizer 4e, Beckman Coulter Inc., Brea, CA, USA) using either a 20 µm or a 30 µm aperture depending on equipment availability. Measurements with the 20 µm aperture were used to determine the droplet concentration for droplet diameters between 0.4 µm and 12.0 µm. Measurements with the 30 µm aperture provided concentration of droplets between 0.6 and 18.0 µm. Coulter counter measurements were made from a stock solution of droplets on the day of the ADV experiments to determine droplet size and concentration. No significant differences in oxygen scavenging were observed when using either aperture to estimate droplet concentrations used in the reported experiments.
Scavenging oxygen from Krebs-Henseleit buffer and whole blood with acoustic droplet vaporization
The ultrasonic core of an EKOS catheter with a 6 cm treatment zone (Model 500-56106, Boston Scientific, Inc., Natick, MA, USA) was inserted into the 106 cm, 5.4F infusion catheter and threaded into the flow phantom tubing ( Fig 1 ). Insonation parameters used in all experiments were based on the work of Benton et al. [ ]: center frequency of 2.35 MHz, 40 cycles, 1.000 ms burst period and time-average electrical drive power ranging between 0.795 W and 0.800 W (corresponding with a pulse average power of 46.7–47.0 W and a peak rarefactional pressure at the exterior surface of the catheter over each transducer pair of 1.5 MPa [ ]).
Droplets infused through the EKOS catheter exited into the sample fluid of either bovine whole blood or KHB. Changes in blood pH, temperature, and pCO 2 alter the affinity of hemoglobin to oxygen [ , ]. Thus, the blood was prepared as follows to yield a physiologic response: pH was 7.35–7.45; sO 2 was 95%–100%, pO 2 was 90–110 mmHg and pCO 2 was 30–50 mmHg [ , ]. KHB is often used in experiments for perfusing animal hearts and was, therefore, the buffer choice for experiments reported herein [ , ]. The KHB was prepared by passing it through the membrane oxygenator with an oxygen flow rate between 55 and 70 mL/min to achieve 90–110 mmHg pO 2 .
The sample fluid flow rate was maintained at 13.4 mL/min. When combined with droplets infused through the EKOS catheter at 1.6 mL/min, a total flow rate in the phantom of 15 mL/min was achieved. This flow rate was used throughout all experiments because higher flow rates required increasing the volume of droplets diluted in KHB to be infused through the EKOS catheter and into the sample fluid to maintain target concentrations. For experiments requiring the use of whole blood, increased dilution thereof resulted in inaccurate BG analyzer readings. This consideration also aligned with the coronary flow rates commonly achieved during Langendorff rat heart perfusion [ , ]. Although it would be possible to use whole blood as a droplet diluent for these in vitro studies, KHB was elected as the droplet diluent to enable future translatability of these results to a pre-clinical model. Temperature and pO 2 were monitored continuously with the dissolved oxygen sensors (TOFTC2, PyroScience GmbH, Aachen, Germany). When the fluid reached the targeted pO 2 range, droplets were infused at 1.6 mL/min through the coolant port of the infusion catheter of the EKOS catheter. To achieve target concentrations of 0.5 × 10 −4 , 2.5 × 10 −4 or 5.0 × 10 −4 mL droplets/mL fluid in the flow phantom, the stock droplets were measured. A dilution factor was calculated, which accounted for both an assumed loss of 27% during the droplet infusion (based on pilot experiments) and the relative flow rates of the oxygenated fluid ( Fig. 1 ) and syringe pump used to infuse the droplets. This target concentration range was selected based on the findings by Benton et al. [ ], who determined that concentrations between 0.5 × 10 −4 and 5.0 × 10 −4 mL/mL scavenged between 20% and 65% of available oxygen from a buffer solution. After 2 min of the droplet infusion, the ultrasound core of the EKOS catheter was turned on while continuing to infuse droplets for another 2 min, during which ADV was initiated within the coolant port of the infusion catheter ( Fig. 1 ).
To assess oxygen scavenging in whole blood, it was prepared to mimic physiologic conditions of arterial blood. Blood had been anti-coagulated with citrate phosphate dextrose, which acidifies it by chelating calcium ions [ ]. In clinical practice, heparin is the preferred anti-coagulant for arterial BG analysis [ ]. However, in our hands, only citrate anti-coagulation prevented the formation of small clots in whole blood, which were detrimental to the BG analyzer function. Therefore, to increase the pH of the blood and bring it closer to physiologic levels, 1 mL/eq sodium bicarbonate solution was added before each experiment [ ]. The procedure for oxygen scavenging from whole blood was similar to that used for KHB, except BG analyzer measurements were used instead of the dissolved oxygen sensors owing to the need to directly measure sO 2 and pO 2 simultaneously. Approximately 2 mL of blood were collected into a syringe for each BG analyzer measurement ( Fig. 1 ). One BG measurement was made for blood that passed through the membrane oxygenator, but had no droplets infused to ensure that physiologic conditions of the blood were maintained (herein referred to as a “before droplet infusion” measurement) [ , ]. A second BG measurement was taken from the same location at the end of the 2 min of droplet infusion without ultrasound exposure (herein referred to as a “after droplet infusion” measurement). A third BG measurement was made from the same location but after 2 min of droplet infusion and ultrasound insonation (herein referred to as an “after ultrasound exposure” measurement) [ , ]. The total oxygen scavenging was defined as the difference between pO 2 levels before droplet infusion and after ultrasound exposure. ADV-mediated oxygen scavenging was defined as the difference between pO 2 levels after droplet infusion and after ultrasound exposure.
To compare the magnitude of oxygen scavenging from plasma and hemoglobin, the pO 2 , expressed in mmHg and sO 2 , expressed as a percent of complete hemoglobin saturation, were converted to oxygen moles per unit volume of fluid. pO 2 was converted using Henry’s law using Henry’s constants specific to each fluid [ ]. Hemoglobin-bound oxygen concentration was determined using the following equation:
CHbO2=CHbtotMHb×(S×4molO21molHb),
C H b O 2
was the concentration of hemoglobin-bound oxygen in moles of oxygen per liter of blood, <SPAN role=presentation tabIndex=0 id=MathJax-Element-6-Frame class=MathJax style="POSITION: relative" data-mathml='CHbtot’>𝐶𝐻𝑏𝑡𝑜𝑡CHbtot
C H b t o t
was the hemoglobin concentration as measured by the BG analyzer in <SPAN role=presentation tabIndex=0 id=MathJax-Element-7-Frame class=MathJax style="POSITION: relative" data-mathml='gL’>𝑔𝐿gL
g L
, <SPAN role=presentation tabIndex=0 id=MathJax-Element-8-Frame class=MathJax style="POSITION: relative" data-mathml='MHb’>𝑀𝐻𝑏MHb
M H b
was the molar mass of hemoglobin (64,500 <SPAN role=presentation tabIndex=0 id=MathJax-Element-9-Frame class=MathJax style="POSITION: relative" data-mathml='gmol’>𝑔molgmol
g mol
), <SPAN role=presentation tabIndex=0 id=MathJax-Element-10-Frame class=MathJax style="POSITION: relative" data-mathml='S’>𝑆S
S
was the sO 2 measured with the BG analyzer and reported as the average fraction of O 2 binding domains filled per hemoglobin molecule, with a fully saturated value of 1 representative of 4 O 2 molecules per 1 hemoglobin molecule.
Determining droplet transition efficiency in the KHB and bovine whole blood
Droplet TE measurements were made using size distributions acquired from either a Coulter counter (Multisizer 4e, Beckman Coulter, Inc.) or spectral flow cytometer (Aurora, Cytek, Fremont, CA, USA). Coulter counter measurements were performed using 200 µL or 15 µL flow phantom samples for experiments. The samples were diluted in 10 mL of sterile-filtered PBS. Flow cytometry data were acquired by setting the microfluidic flow rate to “low” and monitored to stay above 10 µL/s, indicating lack of blockage of the microfluidic line by blood cells or spontaneously forming PFC microbubbles. A 10 µL aliquot of each sample were acquired. All data were acquired with the side scatter (SSC) threshold of 10,000. The forward scatter and SSC voltage gain settings were established with light scatter calibration beads ranging in size from approximately 0.2 µm to approximately 1.0 µm (Rosetta Calibration beads, Exometry, Amsterdam, the Netherlands). One drop of vortexed beads was diluted in 560 µL of sterile-filtered PBS, and data acquired until at least four separate peaks indicating four different bead sizes appeared on the SSC graph [ ]. To identify the fluorescence intensity threshold, 0.5 µL of fluorescent and non-fluorescent stock droplet solutions were diluted in 1 mL of PBS sterile-filtered through a 0.2 µm filter each day to serve as reference controls.
All experiments for determining PFP and DFB droplet transition efficiencies at varying concentrations were performed with fluorescent droplets, because no significant differences in TE of fluorescent and non-fluorescent droplets had been found in pilot studies ( p = 0.719, data not shown). Lipid aggregates or liposomes inadvertently made with DSPC/DSPE-PEG2000 are typically less than 0.2 μm in diameter [ , , ] and were not detected with flow cytometry for our formulation. Samples for TE measurements were collected from the flow phantom in the same manner as described above for oxygen scavenging experiments in whole blood. All TE measurements with the Coulter counter or spectral flow cytometer were performed within 5 h of droplet insonation. Droplets were kept on ice until they were counted.
The TE was determined by diluting 2 µL of each sample collected from the flow phantom (buffer/blood before droplet infusion, after droplet infusion and after ultrasound exposure) in 1 mL sterile-filtered PBS and analyzed on the flow cytometer. Data collected with the SSC parameter were converted to particle diameters using the Rosetta Calibration software (Exometry). The PFC droplets were assumed to be uniform spheres with a core refractive index of 1.44 based on recommendations from Exometry. FlowJo 10.10 (Becton Dickinson, Franklin Lakes, NJ, USA) was used to analyze acquired flow cytometry data. Particles were first gated based on fluorescence intensity. Next particles were gated to either measure particles 0.023 µm or 0.600 µm in diameter and larger. To compare the TE from measured droplet size distributions using both the Coulter counter and the flow cytometry data, the 0.600 µm gated data was used. After obtaining number-weighted size distributions from flow cytometry data, volume-weighted distributions were calculated [ ].
The TE was calculated using the total volume-weighted droplet concentration (i.e., the integral of the volume-weighted size distribution). The difference in the droplet concentration before and after ultrasound exposure was divided by the droplet concentration before ultrasound exposure, per Benton et al. [ ]. The rationale for using volume-weighted size distributions was due to the dependence of oxygen scavenging on the total volume of droplets transitioned [ , ]
Modeling oxygen scavenging via acoustic droplet vaporization from bovine whole blood
Radhakrishnan et al. developed an analytic model that estimated the magnitude of oxygen scavenging in aqueous buffers based on the volume of PFC phase transitioned per unit volume of buffer [ , , ]. The model has three compartments for oxygen (droplets, ADV microbubbles and the buffer). By adding a fourth compartment for hemoglobin, the model was extended to estimating oxygen scavenging in whole blood.
Before vaporization, the oxygen in blood was distributed in the plasma, PFC droplets and hemoglobin compartments. Per the Radhakrishnan model, the moles of oxygen in the plasma and PFC droplets per unit volume were computed from Henry’s law. The moles of hemoglobin-bound oxygen per unit volume of blood was computed based on the hemoglobin concentration and sO 2 . The total number of moles of O 2 in the system before vaporization, <SPAN role=presentation tabIndex=0 id=MathJax-Element-11-Frame class=MathJax style="POSITION: relative" data-mathml='n0′>𝑛0n0
n 0
, was expressed as a sum:
n0=nl0+nd0+nHb0=Pl0Vl0kl+KdPd0Vd0kl+4·CHb0·Vl·S0(Pl0,T,pH),
n l 0 , n d 0
and <SPAN role=presentation tabIndex=0 id=MathJax-Element-14-Frame class=MathJax style="POSITION: relative" data-mathml='nHb0′>𝑛𝐻𝑏0nHb0
n H b 0
are the number of moles of oxygen in the plasma, droplet and hemoglobin compartments before vaporization, respectively. Correspondingly, <SPAN role=presentation tabIndex=0 id=MathJax-Element-15-Frame class=MathJax style="POSITION: relative" data-mathml='Pl0′>𝑃𝑙0Pl0
P l 0
and <SPAN role=presentation tabIndex=0 id=MathJax-Element-16-Frame class=MathJax style="POSITION: relative" data-mathml='Pd0′>𝑃𝑑0Pd0
P d 0
are the pO 2 in the plasma and droplet compartments, respectively. <SPAN role=presentation tabIndex=0 id=MathJax-Element-17-Frame class=MathJax style="POSITION: relative" data-mathml='Vl0′>𝑉𝑙0Vl0
V l 0
and <SPAN role=presentation tabIndex=0 id=MathJax-Element-18-Frame class=MathJax style="POSITION: relative" data-mathml='Vd0′>𝑉𝑑0Vd0
V d 0
are the volumes of the compartments and <SPAN role=presentation tabIndex=0 id=MathJax-Element-19-Frame class=MathJax style="POSITION: relative" data-mathml='kl’>𝑘𝑙kl
k l
and <SPAN role=presentation tabIndex=0 id=MathJax-Element-20-Frame class=MathJax style="POSITION: relative" data-mathml='kd’>𝑘𝑑kd
k d
are the Henry’s volatility constants for these compartments and <SPAN role=presentation tabIndex=0 id=MathJax-Element-21-Frame class=MathJax style="POSITION: relative" data-mathml='Kd’>𝐾𝑑Kd
K d
is the ratio <SPAN role=presentation tabIndex=0 id=MathJax-Element-22-Frame class=MathJax style="POSITION: relative" data-mathml='klkd’>𝑘𝑙𝑘𝑑klkd
k l k d
[ , ]. Following the Radhakrishnan model, the system is assumed to be at equilibrium before vaporization and thus <SPAN role=presentation tabIndex=0 id=MathJax-Element-23-Frame class=MathJax style="POSITION: relative" data-mathml='Pl0=Pd0′>𝑃𝑙0=𝑃𝑑0Pl0=Pd0
P l 0 = P d 0
. <SPAN role=presentation tabIndex=0 id=MathJax-Element-24-Frame class=MathJax style="POSITION: relative" data-mathml='CHb0′>𝐶𝐻𝑏0CHb0
C H b 0
is the hemoglobin concentration, which can be measured from the BG analyzer and <SPAN role=presentation tabIndex=0 id=MathJax-Element-25-Frame class=MathJax style="POSITION: relative" data-mathml='S0′>𝑆0S0
S 0
is the hemoglobin sO 2 , which is dependent on the pO 2 in the plasma, temperature, and pH; per a modified Adair model for bovine blood [ ].
After vaporization, a fourth component, the ADV microbubbles contain oxygen based on the ideal gas law per the Radhakrishnan model:
nb=PbVbRT,
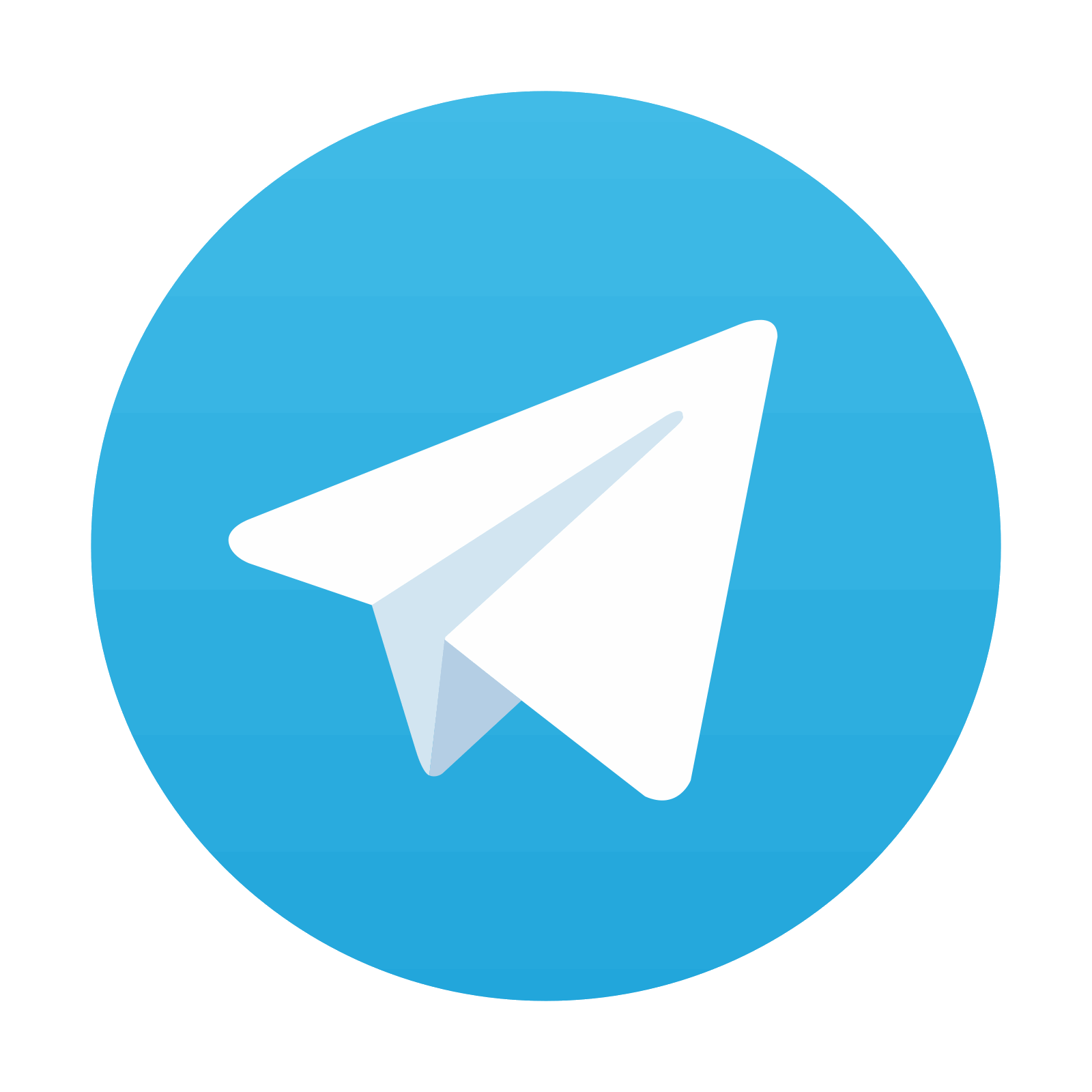
Stay updated, free articles. Join our Telegram channel
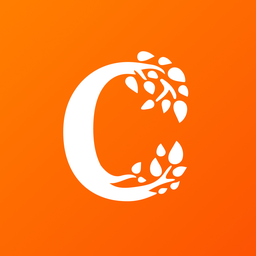
Full access? Get Clinical Tree
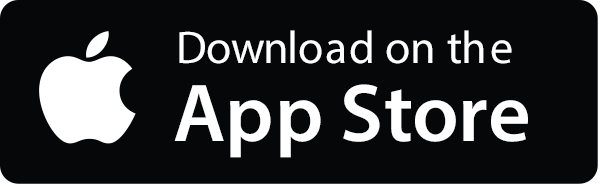
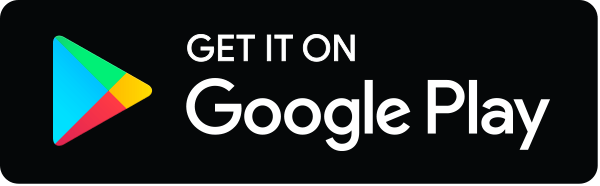
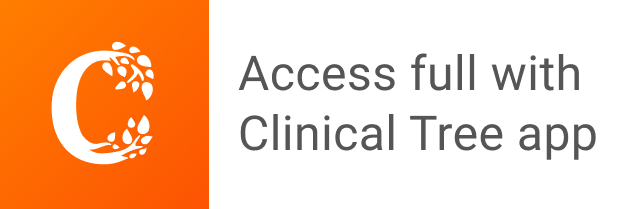