Imaging with X-Rays
An x-ray is a discrete bundle of electromagnetic energy called a photon. In that regard, it is similar to other forms of electromagnetic energy such as light, infrared, ultraviolet, radio waves, or gamma rays. The associated electromagnetic energy can be thought of as oscillating electric and magnetic fields propagating through space at the speed of light. The various forms of electromagnetic energy differ only in frequency (or wavelength). However, because the energy carried by each photon is proportional to the frequency (the proportionality constant is called Planck’s constant), the higher frequency x-ray or gamma ray photons are much more energetic than, for example, light photons and can readily ionize the atoms in materials on which they impinge. The energy of a light photon is of the order of one electron-volt (eV), whereas the average energy of an x-ray photon in a diagnostic x-ray beam is on the order of 30 kiloelectron volts (keV) and its wavelength is smaller than the diameter of an atom (10−8 cm).
In summary, an x-ray beam can be thought of as a swarm of photons traveling at the speed of light, each photon representing a bundle of electromagnetic energy.
Electromagnetic radiation may be produced in a variety of ways. One method is the acceleration or deceleration of electrons. For example, a radio transmitter is merely a source of high-frequency alternating current that causes electrons in an antenna wire to which it is connected to oscillate (accelerate and decelerate), thereby producing radio waves (photons) at the transmitter frequency. In an x-ray tube, electrons boiled off from a hot filament (Figure 2-1) are accelerated toward a tungsten anode by a high voltage on the order of 100 kilovolts (kV). Just before hitting the anode, the electrons will have a kinetic energy in kiloelectron volts equal in magnitude to the kilovoltage (eg, if the voltage across the x-ray tube is 100 kV, the electron energy is 100 keV). When the electrons smash into the tungsten anode, most of them hit other electrons, and their energy is dissipated in the form of heat. In fact, the anode may become white-hot during an x-ray exposure, which is one reason for choosing an anode made of tungsten, with a very high melting point. The electrons penetrate the anode to a depth less than 0.1 mm.
A small fraction of the electrons, however, may have a close encounter with a tungsten nucleus, which, because of its large positive charge, exerts a large attractive force on the electron, giving the electron a hard jerk (acceleration) of sufficient magnitude to produce an x-ray photon. The energy of the x-ray photon, which is derived from the energy of the incident electron, depends on the magnitude of the acceleration imparted to the electron. The magnitude of the acceleration, in turn, depends on how closely the electron passes by the nucleus. If one imagines a target consisting of a series of concentric circles, such as a dart board, with the bull’s-eye centered on the nucleus, more electrons clearly will impinge at larger distances than in the bull’s-eye; hence, a variety of x-ray photon energies will be produced at a given tube voltage (kV) up to a maximum equal to the tube voltage (a hit in the bull’s-eye), where the electron gives up all its energy to the x-ray photon. Increasing the voltage will shift the x-ray photon spectrum to higher energies, and higher-energy photons are more penetrating. The radiation produced in this manner is called Bremsstrahlung (braking radiation) and represents only about 1% of the electron energy dumped into the anode by the electron beam; the other 99% goes into heat.
The electron current from filament to anode in the x-ray tube is called the mA, because it is measured in milliamperes. The mA is simply a measure of the number of electrons per second making the trip across the x-ray tube from filament to anode. The rate of x-ray production (number of x-rays produced per second) is proportional to the product of milliamperage and kilovoltage squared. The quantity of x-rays produced in an exposure of duration s (in seconds) is proportional to the product of mA and time and is called the mAs. The quantity of x-rays at a given point is generally measured in terms of the amount of ionization per cubic centimeter of air produced at that point by the x-rays and is measured in roentgens (R) or in coulombs per kilogram of air. This quantity is called exposure, and 1 R of exposure results in 2 × 109 ionizations per cubic centimeter of air.
The electron beam is made to impinge on a small area on the anode of the order of 1 mm in diameter in order to approximate a point source of x-rays. Because a radiograph is a shadow picture, the smaller the focal spot, the sharper the image. By analogy, a shadow picture on the wall (such as a rabbit made with one’s hand) will be much sharper if a point source of light such as a candle is used rather than an extended light source such as a fluorescent tube. The penumbra (or unsharpness) of the shadow will depend not only on the source size, but also on the magnification, as can be illustrated by making a shadow of one’s hand on a piece of paper using a small light source such as a single light bulb. The closer you bring your hand to the paper (the smaller the magnification), the sharper the edges of the shadow. Similarly, magnification of the x-ray image produced by the point source is less, the closer the patient is to the film and the farther the source is from the film. The magnification factor (M) is defined as the ratio of image size to object size and is equal to the ratio of the focal-to-film distance divided by the focal-to-object distance (M ≥ 1, and M = 1 means no magnification is produced; ie, either the object is right against the film, or the focal spot is infinitely far away). The penumbra, blurring, or unsharpness (Δx) produced on an otherwise perfectly sharp edge of an object and due to the finite focal spot size of dimension a is expressed by the equation
Unfortunately, the smaller the focal spot, the more likely it is that the anode will melt. The power (energy/per second) dumped into the anode is equal to the product of the kilovoltage and milliamperage; ie, at 100 kV and 500 mA, 50,000 watts of heat energy is deposited into an area on the order of a few square millimeters (imagine a 50,000-watt light bulb to get an idea of the heat generated).
X-rays primarily interact with matter through interaction of their oscillating electric field with the atomic electrons in the material. Having no electrical charge, the x-rays are more penetrating than other types of ionizing radiation (such as alpha or beta particles) and are therefore useful for imaging the human body. The x-rays may be absorbed or scattered by the atomic electrons. In the absorption process (photoelectric absorption), the x-ray is completely absorbed, giving all its energy to an inner shell atomic electron, which is then ejected from the atom and goes on to ionize other atoms in the immediate vicinity of the initial interaction. In the scattering process (Compton scattering), the x-ray ricochets off an atomic electron, losing some of its energy and changing its direction. The recoiling electron also goes on to ionize hundreds of atoms in the vicinity. Electrons from both processes go on to ionize many other atoms and are responsible for the biological damage produced by x-rays.
The attenuation of the x-ray intensity with thickness of material follows an exponential law due to the random hit-or-miss nature of the interaction. The process is similar to firing a volley of rifle bullets into a forest, where the bullets may either stick in a tree (be absorbed) or ricochet off a tree (scatter). The deeper you go into the forest, the fewer bullets there are; however, a bullet still has a chance of traveling through the forest without hitting a tree. Likewise, an x-ray can make it all the way through a patient’s body without touching anything and remain unchanged, as if it had passed through a vacuum instead. These are called primary x-rays. Typically, only about 1% of the incident x-rays penetrate the patient, and only about a third of these are primary x-rays; the rest are scattered x-rays that do not contribute to the anatomic image. An x-ray image is a shadow or projection image which assumes that x-rays reaching the film have traveled in a straight line from the source, but this is true only for the primary x-rays. As Figure 2-2 A shows, the film density (blackness) at point P on the film is related to the anatomy along line FP. The scattered photon reaches the film along the path FSP and is relaying information about the anatomy at the random point S to point P on the film. Scatter simply produces a uniform gray background; it does not contribute to the image. Because scatter reduces image contrast, it is desirable that the scatter be removed. This task is accomplished by use of an antiscatter grid (Figure 2-2 B). This grid consists of a series of narrow lead strips with radiolucent (low-attenuation) interspace material to remove some of the scatter. With the grid, the scattered photon shown in the figure can no longer reach the film, but the primary x-rays can. More of the scatter than primary x-rays is eliminated by the grid; hence, image contrast increases, but at the cost of an increase of a factor of 2 to 3 in patient dose. This increase occurs because the scatter, which was previously blackening the film, has been reduced, and therefore, higher x-ray exposure to the front of the patient is necessary to get the requisite number of x-rays through the grid to blacken the film. The grid is usually made to move a few interspaces during the exposure by a motor drive, in order to wash out the grid lines.
The absorption process is more prevalent at lower kilovoltages and in materials with higher atomic numbers. Bones appear white on an x-ray film because photoelectric absorption of x-rays is greater in bone than in soft tissue as a result of the higher atomic number of bone. Lead is a useful shielding material for x-rays because of its high atomic number. The probability of the absorption process decreases rapidly with photon energy (as 1/E3) and the scattering process decreases slowly (as 1/E); hence, the x-ray beam becomes more penetrating as kilovoltage increases. The scattering process is roughly independent of the atomic number of the attenuating material (all electrons look alike to the photon for the scattering process), whereas the absorption process is more probable for tightly bound electrons such as the inner electrons in heavier elements.
Increasing the kilovoltage is therefore beneficial to the patient in that it reduces the radiation dose: that is, fewer x-rays must penetrate the front of the patient to get the requisite number out the back to blacken the film. However, an increase in kilovoltage will reduce image contrast because the absorption process, which is sensitive to atomic number, will decrease and the scattering process is independent of the atomic number of the materials. Even with materials of the same atomic number, contrast improves at lower kilovoltage settings because of higher attenuation, which results in greater differential attenuation between different thicknesses of the same material. Thus there is a tradeoff between image quality (contrast) and patient dose that must be weighed in the selection of kilovoltage.
For production of radiographic images, the x-ray film is placed in a cassette and sandwiched between two fluorescent screens that glow under x-ray exposure, and it is primarily the light from these fluorescent screens that blackens the film. Although x-ray film, which is quite similar to ordinary photographic film, can be blackened by direct x-ray exposure, the film does not absorb the penetrating x-rays very efficiently, because the emulsion consists of silver halide crystals embedded in a low-atomic-number gelatin base. The fluorescent screens, called intensifying screens, are made of high-atomic-number materials, which therefore absorb x-rays very efficiently and also emit hundreds of light photons per x-ray absorbed. These light photons, in turn, are efficiently absorbed by the film. As a result, x-ray exposure to the patient is reduced by a factor on the order of 100 compared to direct x-ray exposure of the film. The screens do produce a loss of sharpness of the image due to the spreading out of the light from the point of x-ray absorption before the light reaches the film. This effect can be reduced by making the screen thinner; however, it then absorbs a smaller fraction of the incident x-rays and therefore results in a “slower” system (more patient exposure is required).
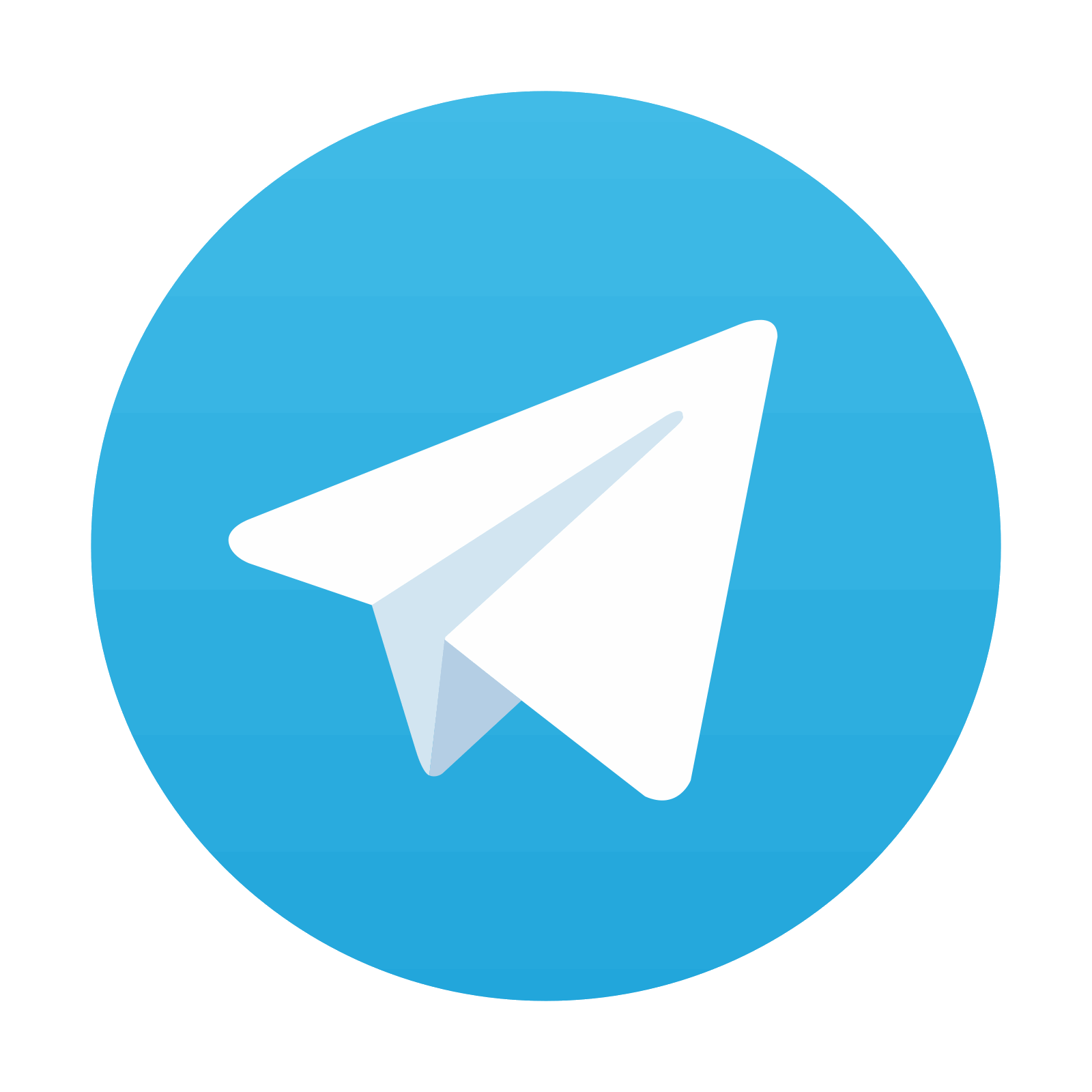
Stay updated, free articles. Join our Telegram channel
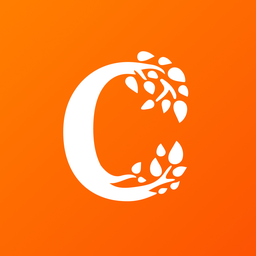
Full access? Get Clinical Tree
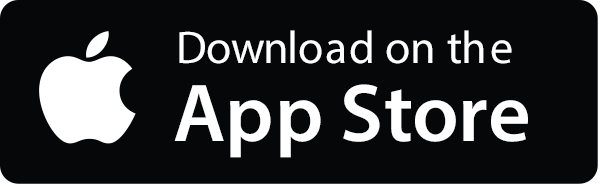
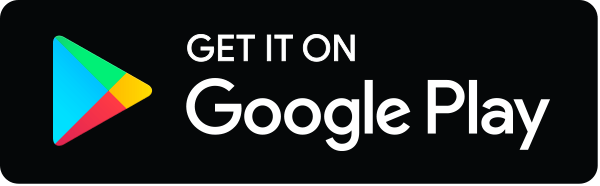