Abstract
Objective
Acoustic clutter is a major source of image degradation for in vivo ultrasound imaging. However, clutter is often not represented in laboratory testing of ultrasound equipment. A phantom material is proposed that can be used to add calibrated amounts of clutter in the laboratory environment.
Methods
Previously, the speed of sound in agar has been adjusted by varying the concentration of propanol to which the agar is exposed. That property was leveraged in this work to create a phantom with an adjustable amount of clutter. Agar spheres were soaked in propanol solution, then strained and placed in mineral oil.
Results
Image quality measurements showed an approximate range of achievable contrast degradation levels of 15 dB. Stability studies with the phantom material showed that it can be stored for at least 21 d after the speed of sound tuning in propanol, but once introduced to mineral oil the clutter will change over time.
Conclusion
This work demonstrates a clutter-generating phantom material that can be used in conjunction with standard ultrasound imaging phantoms.
Introduction
Ultrasound phantoms are routinely used to evaluate ultrasound imaging systems. In research environments phantoms are used to assess new imaging sequences and beamforming methods, whereas in clinical environments phantoms are used for quality assurance. In each case, embedded targets with known properties, such as point targets or cysts of known size and contrast, are used to validate system performance [ , ]. Phantoms are produced from a variety of materials and range in structure from simple blocks to anthropomorphic phantoms that mimic organ systems [ ]. When designing phantoms, care is taken to ensure that the acoustic properties of the material are tissue-mimicking by matching the speed of sound, attenuation, scattering properties and frequency response observed in vivo [ , ]. However, these properties are often only accurate on average over large spatial extents and imaging phantoms fail to reproduce the fine-scale noise-generating acoustic heterogeneities that degrade in vivo imaging. In this work, a tunable, acoustic clutter-generating layer is demonstrated to introduce clinically relevant imaging conditions to a phantom environment.
Acoustic clutter, a temporally stable noise that appears in ultrasound images as a speckle-like haze, obscures target features and degrades contrast [ ]. The loss in contrast between targets can be up to 45 dB in vivo [ ]. Despite the prevalence of clutter in clinical imaging, it is often absent in phantom imaging. Tissue heterogeneities have long been understood as the source of reverberation and aberration clutter. In the case of reverberation, reflections between structures of dissimilar acoustic impedance and multiply-scattered wavefronts result in signals received by the transducer being overwritten by undesired echoes from disparate spatial locations [ ]. Phase aberration is caused by differences in the expected acoustic path length between the transducer elements and a target due to heterogeneity in acoustic velocity [ , ]. In phantoms, the acoustic impedance and velocity are often homogeneous, apart from slight variations from embedded targets, so the amount of aberration and reverberation is limited. As such, phantom imaging tends to replicate only high-quality imaging conditions and contains low levels of temporally stable noise. This is not representative of the typical in vivo environment, where low image quality is a pervasive issue that can lead to errors in clinical diagnosis [ ]. Introducing realistic signal-to-clutter levels in a phantom environment would enable more thorough evaluation of new ultrasound devices or processing techniques at an early stage in the development process, before clinical tests might be feasible.
Ideally, a laboratory clutter-generating mechanism should interface with existing phantoms in order to provide the option of adding clutter to the current research and clinical phantom environment. Additionally, the clutter generated should be tunable to different signal-to-clutter levels to enable imaging under a variety of conditions. Furthermore, these clutter levels should be calibrated and reproducible, allowing for standardized testing and evaluation in different locations and at different times. Physically, the noise produced should come from both aberration and reverberation to match the characteristics of the noise observed during in vivo imaging.
Several techniques have been proposed or used to meet these aims, although all fall short on one or more counts. A natural laboratory test bed for ultrasound imaging is ex vivo tissue. The layers of fat, muscle and connective tissue present in ex vivo tissue produce clutter from aberration and reverberation, generating a relevant imaging environment for pre-clinical studies [ , ]. While the clutter-generating method of ex vivo specimens follows the same mechanisms present during in vivo scanning, the main drawback to this technique is that it is not reproducible between labs or at different times due to variation across specimens and degradation of the tissue. Ex vivo tissue is also not calibrated to test performance under specific signal-to-clutter ratios. Simulations of tissue models can attempt to correct for these drawbacks [ , ], but verifying the tissue models used, as well as the characteristics of the simulation environment, can prove difficult.
Manufactured clutter-generating mechanisms offer an alternative approach. Electronic channel delays can be introduced at transmit or receive to simulate the effects of aberration [ ], but this technique does not replicate the spatially distributed origin of aberration nor does it incorporate the effects of reverberation. Alternatively, molded layers with different thicknesses, shapes and speeds of sound can be introduced [ , ]. This option is both tunable and reproducible, but the layers of these phantoms typically involve sharp boundaries that do not faithfully reproduce the irregularity within and between layer interfaces. An oil-in-gelatin emulsion phantom was created by Lacefield et al. to introduce aberration to laboratory environments, but a means of tuning to specific desired signal-to-clutter levels was not demonstrated [ ].
Here, we propose a mechanism for introducing clutter to existing phantoms in a way that is tunable, reproducible and representative of clutter observed in vivo . Agar has been used as a tissue-mimicking material for many years [ ], and it can match the speed of sound, density and attenuation co-efficient of soft tissue [ ]. Building from this previous work, agar material can be combined with an oil-based interstitial fluid to mimic the cluttered environment of in vivo ultrasound scanning. When this material is placed on top of existing ultrasound phantoms, clutter overwrites the underlying targets. Temporally stable acoustic noise is generated from reverberation within and aberration through agar spheres immersed in a mineral oil solution. Calibrated adjustment of the acoustic characteristics of the agar spheres can be performed, and the resulting effect on image quality has been observed.
This article demonstrates the feasibility of an agar-based, tunable clutter-generating phantom. First, the achievable sound speeds of the agar spheres are presented. Then, the method of generating clutter is introduced. Quantitative and qualitative imaging results are shown using this novel material over a standard ultrasound phantom with anechoic cysts. Additionally, results are shown for stability tests of the clutter-generating phantom material. Potential applications of this type of phantom and future directions of the work are discussed.
Materials and methods
In this work, a clutter-generating ultrasound phantom material was produced that mimics in vivo imaging conditions in a laboratory environment. This method uses agar spheres as a base and allows the amount of clutter generated to be adjusted to a range of clinically relevant levels. When the agar spheres are soaked in different concentrations of 1 -propanol, they achieve different speeds of sound. These spheres are then strained and placed in a mineral oil solution. The resulting semi-liquid phantom can be placed in a container on top of commercially available phantoms to create degraded laboratory imaging conditions that better match the reality of ultrasound use in a clinical environment.
Tuning the speed of sound of agar spheres
The first step in the production of the clutter-generating phantom material is adjusting the speed of sound of the agar spheres to the desired level. The spheres can reach a range of sound speeds depending on the propanol concentrations they are stored in. By straining the spheres and replacing the solution several times, osmosis forces the spheres to come to equilibrium with the solution they are stored in. This can, theoretically, be adjusted from pure water to pure alcohol concentrations. Liquid Germall Plus (Lotioncrafter, Eastsound, WA, USA) is also included in the propanol solutions to serve as an anti-microbial preservative for the phantom material.
Preparation. Agar spheres slurry material (University of Wisconsin, Madison, WI, USA) was used in this experiment. The agar spheres were composed of 3% (by weight) agar and 6% (by weight) talc and were stored in a 10% (by volume) 1-propanol in deionized water solution. The spheres were manufactured using a shot tower and filtered to retain spheres with an approximate diameter range of 0.6–2.5 mm [ ].
To change the speed of sound, the interstitial fluid was replaced with varying concentrations of 1-propanol (0%, 2.5%, 5%, 7.5%, 10%, 12.5%, 15%, 20% and 25%) by volume, 1.5% Liquid Germall Plus by volume and deionized water. The agar spheres slurry material was strained with a cheese cloth. Then, they were divided into jars with 60 g spheres each and filled with 120 mL of the appropriate propanol-based solution. Three samples were created for each of the propanol concentrations. The spheres were strained and the propanol solution was replaced after 2 d. Then, after 2 additional days, the first speed of sound measurements were performed. This was considered day 0.
Speed of sound testing. For speed of sound measurements, the spheres in propanol solution were transferred to a 3D-printed box with a metal plate across the bottom that created a strong reflection. Imaging was performed with a Verasonics Vantage 256 research ultrasound system (Verasonics, Kirkland, WA, USA) and a P4-2v transducer, which has a frequency range of approximately 2–4 MHz.
The transducer began at the top surface of the clutter phantom material and was lowered in 2 mm increments by a XPS-Q8 motion controller (Newport Corporation, Irvine, CA, USA) to collect plane wave images at nine axial locations. Normalized cross correlation between neighboring frames was performed to determine the time shift, and the median shift was used to calculate the speed of sound. Five repeat measurements were performed for each batch of agar spheres. These measurements were made at multiple time points (days 0, 2, 7, 14 and 21) to establish the stability of the spheres in propanol solution.
Transition to clutter-generating material
After the speed of sound was adjusted, the spheres were drained and placed in a mineral oil solution. Imaging through this material produces clutter that overwrites the echoes from underlying structures. Contrast [ ] and contrast-to-noise ratio (CNR) [ , ] measurements were used to quantify the image quality degradation associated with each propanol concentration. Figure 1 shows an overview of the process to make this clutter-generating phantom material.
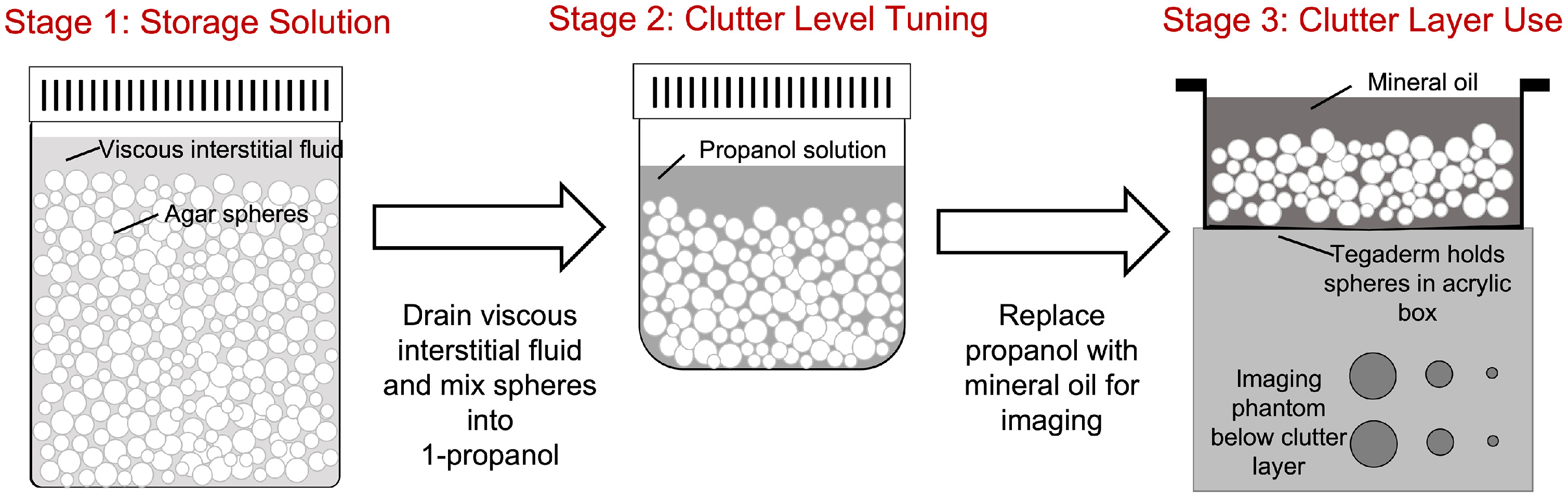
Preparation. Agar sphere slurry material (University of Wisconsin, Madison, WI, USA) known as ‘thermal/acoustic tissue-mimicking slurry with viscous interstitial fluid’, which is commonly used for transducer surface temperature testing in accordance with IEC 60601-2-37 [ ], was used in this experiment. The agar spheres had the same composition as those used in the speed of sound testing experiment described in the ‘Tuning the speed of sound of agar spheres’ section, but in this version they were stored in a solution composed of 30% by weight propylene glycol in deionized water with 2% Grindsted Carrageenan CP 102 FLX (Danisco Chile S.A., Pargua, Chile) and 1.5% Liquid Germall Plus (Lotioncrafter, Eastsound, WA, USA), both by weight. This is referred to as stage 1 in Figure 1 .
To use this slurry material as a phantom, the interstitial fluid was again replaced with a propanol-based solution (stage 2). Based on the results of speed of sound testing, the concentrations of propanol were limited to 0%, 2.5%, 5%, 7.5%, 10%, 12.5% and 15% by volume in a solution with 1.5% Liquid Germall Plus by volume and deionized water. The spheres stored in the viscous solution were strained with a sieve to minimize mechanical damage. Then, they were divided into jars with 75 g of spheres each and filled with 150 mL of the appropriate propanol-based solution. Three samples were created for each of the propanol concentrations. The propanol solution was strained from the spheres and replaced five additional times with 2 d between each swap. Because these spheres were originally stored in a viscous solution, more replacements of the interstitial fluid were performed to wash the spheres and allow the propanol solution to leach in. Intermittent daily mixing was preformed to distribute the fluid around the spheres. A total of 2 d after being placed in the final batches of propanol solution, the jars of spheres were strained and 60 g of spheres were measured and placed in 120 mL of mineral oil with 1.5% (by volume) of Liquid Germall Plus. Another 2 d after the spheres were transferred to the oil solution, day 0 measurements of the clutter-generating phantom material were performed (stage 3).
Image quality testing. To measure image quality, the spheres were placed in a box with acrylic sides and a Tegaderm (3M, St. Paul, MN, USA) base. The box was placed on top of a Model ATS 549 General and Small Parts Phantom (CIRS, Norfolk, VA, USA) with a thin layer of water coupling the Tegaderm and the phantom surface. As shown in Figure 2 , the transducer, attached to a translation stage, was positioned 1 cm above the Tegaderm surface. A region of the phantom with a vertical column of 8 mm diameter cylindrical cysts was imaged through the 1 cm layer of agar spheres. The transducer was moved in the elevation direction, which corresponds to the longitudinal dimension of the cylindrical cysts, in 0.5 cm increments to achieve five different speckle realizations for each batch of spheres. Images were collected with focal depths of 3, 5 and 7 cm, which correspond to the cyst locations, and images were acquired at a transmit frequency of 3 MHz.
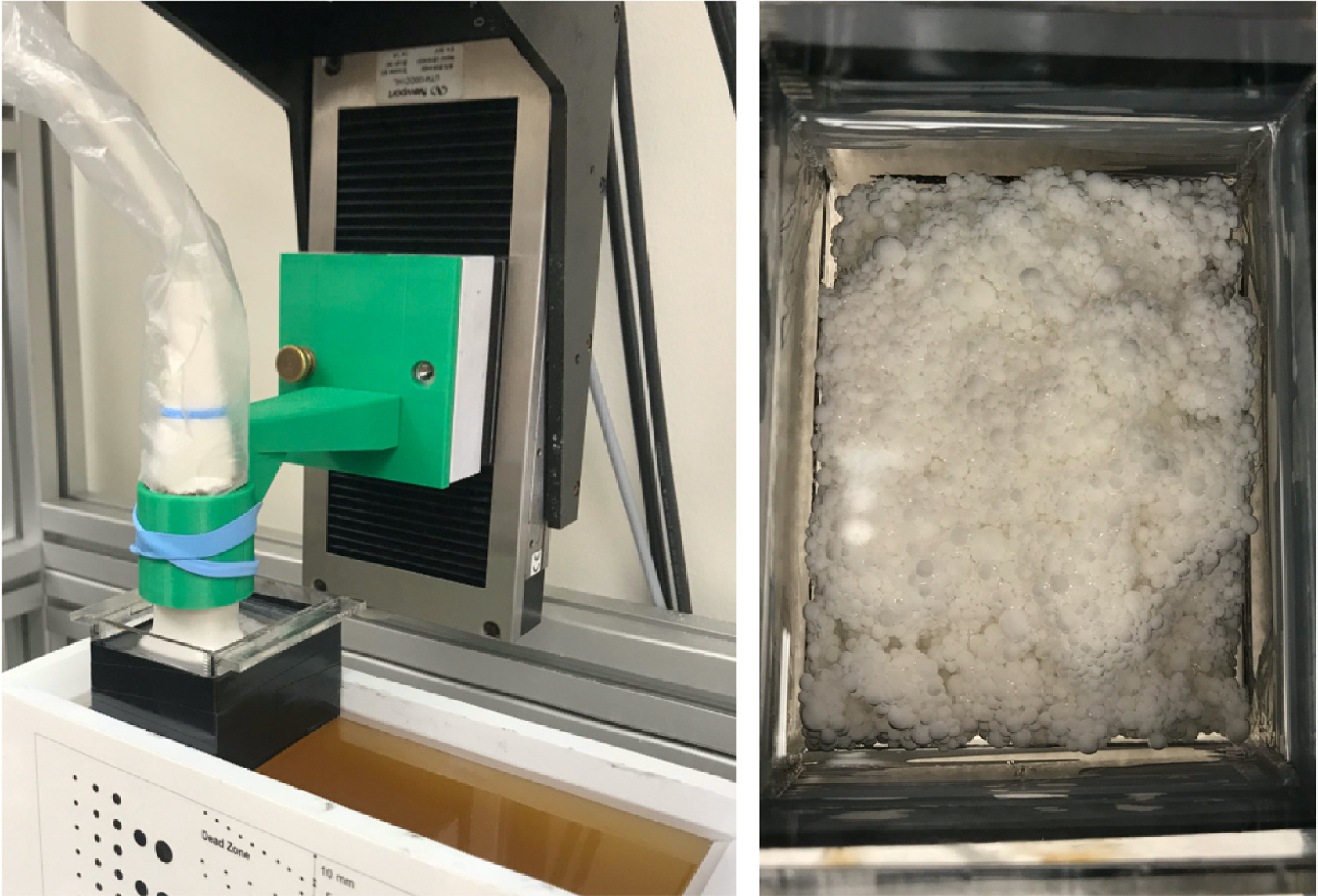
The phantom material was poured into the acrylic boxes at least 12 h before measurements were preformed to allow the spheres to settle. In order to minimize handling of the spheres, the material was left in the boxes and covered with plastic wrap between measurements through day 28, unless there was visible oil leaking. In that case, boxes were replaced. Additionally, mineral oil solution was added to boxes as needed to keep the spheres fully covered. After day 28 measurements, the spheres were removed from the boxes and stored in the jars until they were set up for day 50 measurements.
To evaluate the amount of clutter generated, contrast [ ] and CNR [ , ] were calculated based on the ATS 549 Phantom anechoic cysts and adjacent homogeneous speckle regions. Images were collected on days 0, 2, 7, 14, 21, 28 and 50, and image quality results were compared over time to evaluate the stability of the spheres when stored in the mineral oil solution.
Potential for re-use of clutter-generating phantom material
After evaluating the image quality produced by the clutter-generating layer through time, the potential for re-use of these spheres was investigated, a process we refer to as ‘rejuvenation’. The agar spheres were re-mixed with new solutions of their respective propanol concentrations, again using several replacements of the propanol solution to wash the spheres and allow them to reach equilibrium. Then, the spheres were again placed in a mineral oil solution for use as a clutter-generating source in phantom imaging. The goal of this rejuvenation process was to evaluate the degree to which clutter-generating properties may be recovered after use.
Preparation. Following a similar procedure to the experiment described in the ‘Transition to clutter-generating material’ section, the spheres stored in mineral oil were strained and placed in propanol solutions. Then, the propanol solution was swapped five times with the solution replaced every 2 d. Finally, the spheres were strained and placed in mineral oil with 1.5% (by volume) of Liquid Germall Plus.
Testing. Image quality measurements similar to those described in the ‘Transition to clutter-generating material’ section were made 2 d after transfer to a mineral oil solution (day 0).
Results
Speed of sound
The measured speed of sound for the agar sphere mixtures at varying propanol fractions is shown in Figure 3 a. The speed of sound increased linearly up to a propanol fraction of 0.15, before reaching a plateau and then slightly decreasing at 0.25. To evaluate the strength of this relationship, a Pearson’s correlation coefficient was calculated between the measured sound speed and the concentration up to 0.15. A correlation coefficient value of R 2 = 0.99 indicated a strong relationship between these factors. The propanol fractions used in the remainder of the experiments were limited to the observed linear range of up to 0.15 propanol concentration. Figure 3 b shows the stability of the measured speed of sound over 21 d for four different propanol fractions within this linear range. Separation in speed of sound between samples prepared with different propanol concentrations was maintained throughout the time period investigated. The difference in sound speed observed between concentrations was greater than the standard error of sound speed within a given concentration or the measured sound speed fluctuation across the time series.
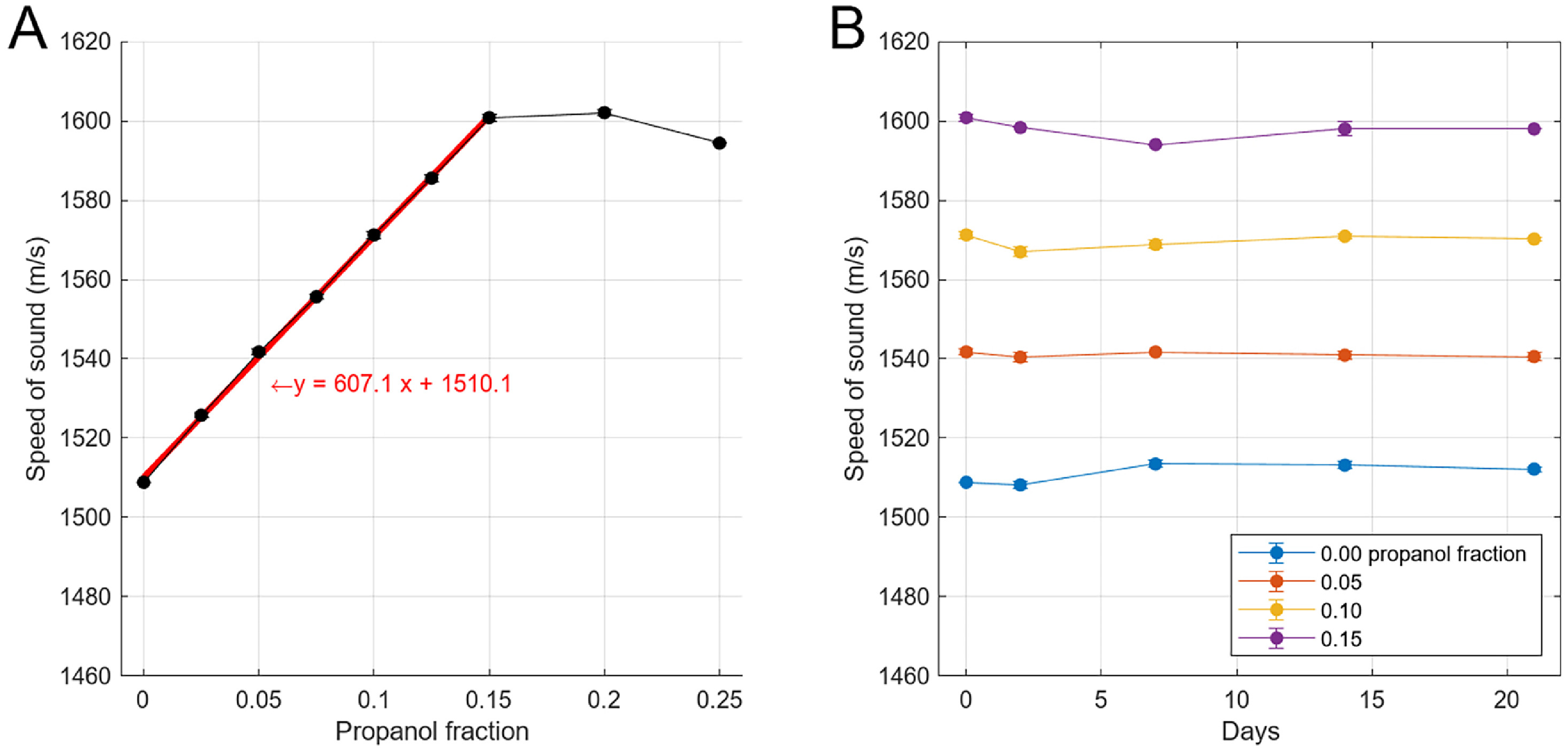
Image quality metrics
After speed of sound adjustments, the spheres were transferred to a mineral oil solution to create the clutter-generating layer. Example images of clutter generation at two depths, 5 and 7 cm, are shown in Figure 4 . To facilitate comparison of the images from each focal depth, they were histogram matched based on regions of homogeneous speckle using the method implemented by Bottenus et al. [ ]. For both focal depths, the 0.00 propanol concentration case, shown in the top row, had the highest perceived image quality. As the concentration of propanol increased to 0.15, image quality decreased until the phantom cysts were completely obscured. In these example images, the transition point from detectable to undetectable was about 0.10 and 0.125 propanol concentration for the 5 and 7 cm focal depths, respectively.
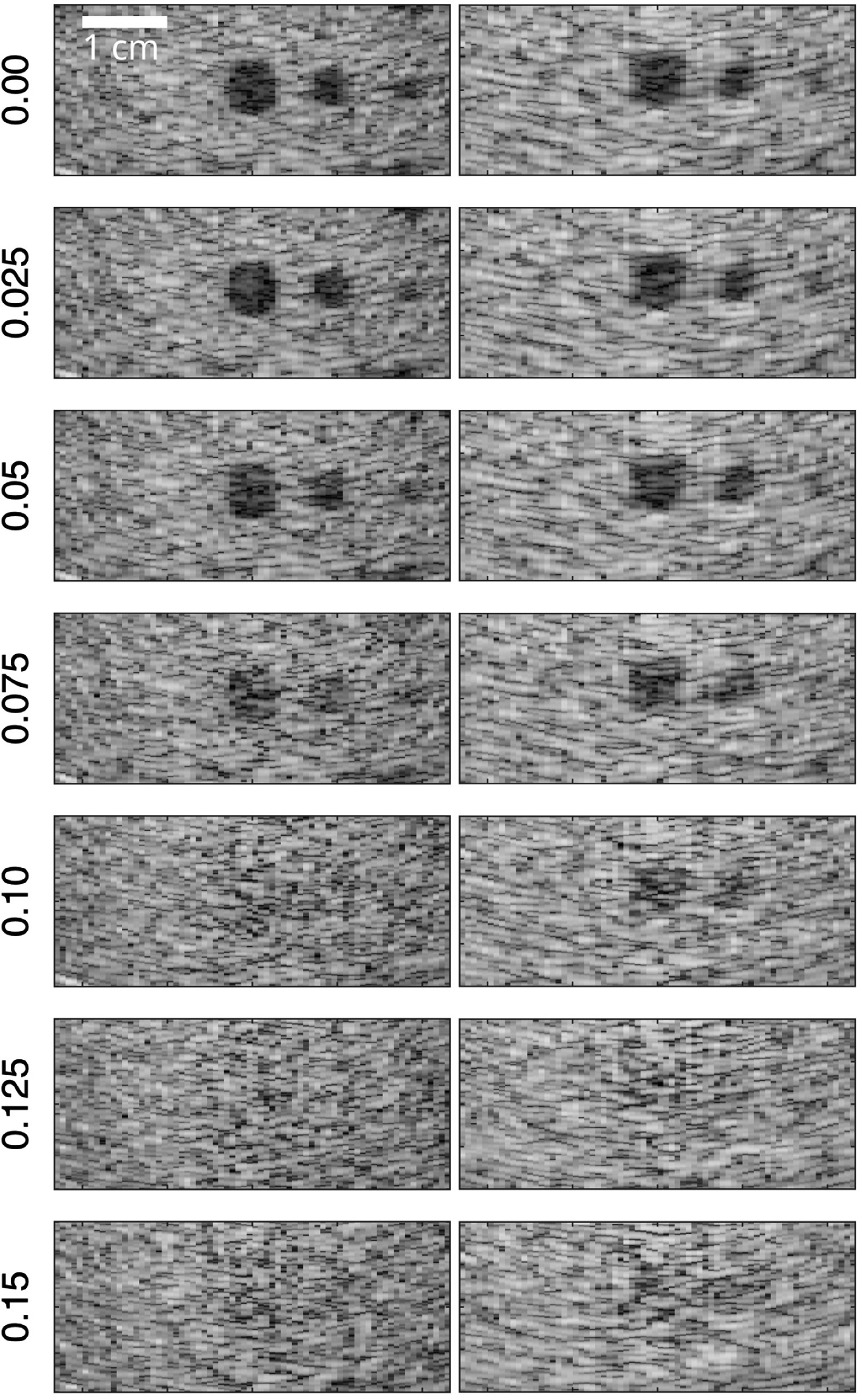
Trends in contrast with propanol fraction are shown in Figure 5 a for cysts at 3, 5 and 7 cm depth. For all three focal depths, contrast decreased monotonically with propanol concentration and approached 0 dB, i.e. , no contrast, at high concentrations. Analogous trends in CNR are shown in Figure 5 b. CNR decreased monotonically with propanol concentration, approaching 0. Contrast and CNR values measured at 5 and 7 cm depths were similar, while contrast and CNR measured at 3 cm were approximately 5 dB and 0.5 lower, respectively.
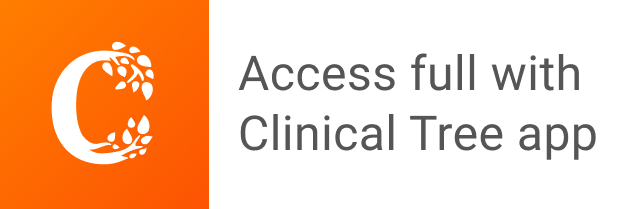