Abstract
Objective
Advanced imaging methods are crucial for understanding stroke mechanisms and discovering effective treatments to reduce bleeding and enhance recovery. In pre-clinical in vivo stroke imaging, MRI, CT and optical imaging are commonly used to evaluate stroke outcomes in rodent models. However, MRI and CT have limited spatial resolution for rodent brains, and optical imaging is hindered by limited imaging depth of penetration. Here we introduce a novel contrast-enhanced ultrasound imaging method to overcome these challenges and characterize intracerebral hemorrhage with unique insights.
Methods
We combined microbubble-based ultrasound localization microscopy (ULM) and nanodrop (ND)-based vessel leakage imaging to achieve simultaneous microvascular imaging and hemorrhage detection. ULM maps brain-wide cerebral vasculature with high spatial resolution and identifies microvascular impairments around hemorrhagic areas. NDs are sub-micron liquid-core particles that can extravasate due to blood-brain barrier breakdown, serving as positive contrast agents to detect hemorrhage sites.
Results
Our findings demonstrate that NDs could effectively accumulate in the hemorrhagic site and reveal the location of the bleeding areas upon activation by focused ultrasound beams. ULM further reveals the microvascular damage manifested in the form of reduced vascularity and decreased blood flow velocity across areas affected by the hemorrhagic stroke.
Conclusion
The results demonstrate that sequential ULM combined with ND imaging is a useful imaging tool for basic in vivo research in stroke with rodent models where brain-wide detection of active bleeding and microvascular impairment are essential.
Introduction
Intracerebral hemorrhage (ICH) represents more than 10% of the 17 million occurrences of stroke globally each year. It is associated with a mortality rate exceeding 40%, and only 20% of the survivors achieve functional independence within 6 mo [ , ]. ICH stands as the second most frequent and most lethal form of stroke, yet effective medically or surgically proven treatments remain elusive [ ]. Due to blood rupture and bleeding into the brain parenchyma, hemorrhages usually lead to an increasing cerebral pressure and subsequent hypoperfusion [ ]. Early detection of alterations in cerebral blood flow (CBF) and microcirculation can provide valuable insights into the underlying mechanisms of hemorrhage. Impairments in microcirculation can result in altered microvascular perfusion and tissue oxygenation, potentially leading to tissue damage and neurological disorders. While CT and MRI are powerful imaging modalities for human and large animal studies, their spatial resolution is insufficient for capturing the microvascular range, making direct measurement of microvascular density and hemodynamics unfeasible [ ]. As such, there is a strong pre-clinical need for whole-brain high-resolution imaging techniques that can provide detailed insights into blood flow and the dynamics of hemorrhage at the microvascular level.
Ultrasound imaging offers an enticing solution for this clinical need because it is widely available in both clinical and non-clinical settings. Ultrasound (e.g., transcranial Doppler) is regularly utilized in the clinic for characterizing major cerebral vessels [ ] but conventional techniques lack sufficient spatial resolution for detecting small vessel abnormalities and also lack extravascular imaging capabilities for detecting vessel leakage.
Ultrasound localization microscopy (ULM) is an emerging super-resolution ultrasound imaging technique that utilizes the localization and tracking of intra-vascular-injected microbubbles (MBs) to image microvessels in deep tissues [ , ]. In addition to structural imaging of microvessels, ULM also leverages MB tracking to measure blood flow velocity [ ]. Previous work by Chavignon et al. [ ] used ULM to characterize ischemic and hemorrhagic stroke in a rat model and demonstrated the effectiveness of using ULM for detecting microvascular perfusion losses during acute onset of stroke. ULM was also used in humans for stroke imaging by Demené et al. [ ], who demonstrated the feasibility of detecting deeply seated aneurysms in stroke patients with transcranial ULM. One common limitation associated with these pioneering works is the use of MBs as contrast agent for stroke imaging [ , ]. Contrast MBs are micron-sized gas-core spheres that are too large to extravasate to provide vessel leakage measurements commonly associated with hemorrhagic stroke. Although quantitative microvascular diffusion indices may be derived to differentiate hemorrhagic versus ischemic stroke, they require prolonged imaging and therefore do not facilitate early detection. As such, a method that offers both direct assessment of vessel leakage and microvascular impairment associated with hemorrhagic stroke remains elusive.
Phase-changing nanodrops (NDs) offer a promising solution for detecting active bleeding in hemorrhagic stroke due to their smaller size and longer circulation time than MBs [ ]. Their reduced size allows them to evade filtration by macrophages in both the cardiopulmonary circulation (e.g., lungs), and the systemic circulation (e.g., liver, and spleen), enhancing their effectiveness in prolonged circulation [ ]. NDs were previously used for ULM applications to enhance microvasculature imaging performance due to their smaller size, which facilitates their perfusion into smaller vessels [ , ]. Upon acoustic activation, non-echogenic NDs expand into echogenic MBs to provide adequate backscattering for detection [ , ]. NDs have also been used in cancer applications to provide vessel leakage measurements, as well as serving as a vehicle for drug delivery [ , ]. Inspired by the existing work and the unique features of NDs, here we propose to combine ND-based vessel leakage imaging with ULM to provide direct and comprehensive evaluation of microvascular impairment associated with stroke. Unlike MBs, NDs have the capability to extravasate from the vasculature. Their small size and perfluorocarbon liquid core facilitate a long circulation half-life, allowing ample time for NDs to extravasate. In addition, owing to their small size, a higher ND count can be delivered than MBs at the same particle volume, which effectively enhances the concentration of NDs in the bloodstream to increase overall extravasation. These advantages make NDs ideal candidates for vessel leakage detection in the context of hemorrhagic stroke.
In this study, the efficacy of ND imaging was initially assessed in a tissue-mimicking flow phantom. A hemorrhagic stroke mouse model based on collagenase injection was subsequently developed to evaluate the feasibility of using NDs for detecting early-stage active bleeding. Concurrent ULM imaging was used to monitor alterations in microvascular density and flow velocity post-hemorrhagic stroke. The combined utility of ULM and ND imaging shows great potential for improving the understanding of stroke pathology through detailed examinations of vascular integrity and blood leakage dynamics.
Methods
Phospholipid NDs preparation
NDs were fabricated at the University of Colorado Boulder using a previously described MB-condensation method [ ]. In brief, lipid films were prepared by dissolving DSPC and DSPE-PEG2K (Avanti Polar Lipids, AL, USA) in a 9:1 molar ratio in chloroform (Sigma-Aldrich, MO, USA), followed by solvent evaporation under nitrogen gas. The films were dried overnight under vacuum and stored at −20°C. Next, the lipid films were rehydrated with 1X PBS to a lipid concentration of 2 mg/mL, heated to 55°C, and sonicated in a water bath at 45°C until transparent. The suspension was cooled to 4°C and sonicated with a Branson 450 Digital Sonifier (Branson, CT, USA) under a perfluorobutane (FluoroMed, TX, USA) hood to generate MBs. The MBs were size-isolated by centrifugation to remove those with diameters greater than 3 µm and stored in crimp-sealed 2-mL serum vials [ ]. To form droplets, the MB suspension was rapidly cooled in 70% isopropyl alcohol solution (Sigma-Aldrich, MO, USA) at −20°C for 2–3 min with periodic mixing. Vials were then pressurized with room-temperature perfluorobutane until the suspension turned transparent, indicating successful gas core condensation. The resulting NDs were transferred to syringes and maintained at 4°C during shipping to UIUC. ND size was assessed using NanoSight NS300 (Malvern Panalytical, Malvern, UK) and Coulter Mutisizer 4e (Beckman Coulter, CA, USA).
Flow phantom experiments
ND activation was verified using a flow phantom with channel’s diameter approximately 450 μm. Ultrasound imaging was conducted using a Verasonics Vantage 256 System (Verasonics, WA, USA) equipped with an L22-14vX, 128-element high-frequency linear transducer, operated at a center frequency of 15.625 MHz (Verasonics, WA, USA). Images were acquired using plane-wave compounding with steering angles ranging from –5 to 5 degrees in 1-degree increments, achieving a post-compounding frame rate of 1,000 Hz. The ND concentration was diluted to 5 × 10 8 particles/mL and introduced into the flow phantom with a 1-mL syringe.
Animal preparations
All animal procedures followed the University of Illinois IACUC guidelines (Protocol #22033). Twelve-week-old female C57BL/6 mice were used for the hemorrhagic model and ND imaging ( n = 3 for each group). Mice were anesthetized with 4% isoflurane in 100% oxygen for induction, followed by 1%–2% for maintenance. The head was secured in a stereotaxic frame (Kopf, CA, USA), and body temperature was maintained at 37°C with a heating pad (Physitemp, NJ, USA). The scalp was removed, and temporalis muscles were dissected. A bilateral craniotomy window was created using a high-speed drill (Foredom, CT, USA). Artificial cerebrospinal fluid (aCSF) was applied to keep the brain tissue moist [ ].
Hemorrhagic stroke mouse model establishment
An automatic nanoinjector (Nanoject II, Drummond, PA, USA) was mounted on a stereotaxic frame via a micromanipulator. A capillary glass needle (Borosilicate, 1.2 × 0.69 mm × 10 cm, Sutter Instruments, CA, USA) filled with mineral oil (Sigma-Aldrich, MO, USA) was inserted into the nanoinjector ( Fig. 1 a). Following this setup, a collagenase solution (0.075 U/0.4 µL in saline) (C0773, Sigma-Aldrich, MO, USA) was drawn into the needle, ensuring no air bubbles. Collagenase, commonly used to establish ICH rodent models [ , ], was injected after craniotomy. The needle was aligned with the bregma, moved 2 mm left and 2 mm caudally, and injected with 100 nL at 1.2 mm depth or 25 nL at 0.6 mm depth. Once both the tail vein needle and the capillary glass needle were in position, the ND infusion commenced for 2 min, followed by the collagenase injection. The needle remained in place for 5 min to ensure collagenase deposition ( Fig. 1 a).

ND activation sequence
NDs were administered via a 29-gauge tail vein catheter with a syringe pump (NE-300, New Era, NY, USA) infusing 1 mL of NDs at 200 µL/min ( Fig. 1 a). During the infusion, physiological parameters including breathing rate, heart rate and body temperature, were closely monitored for signs of physiological stress. After infusion, the mouse remained under anesthesia for 2 h to allow ND extravasation and clearance from the blood vessels. ND imaging involved a special sequence: low-voltage (6V) plane wave for baseline imaging, high-voltage (30V) sweeping focused beams for ND activation (40 cycles at 4 mm focal depth, Fig. 2 ), and another low-voltage sequence for post-activation imaging ( Fig. 1 b). The derated mechanical index (derated MI 0.5 ) (0.5 dB/cm/MHz) of the center focused beam was measured at 0.54, and the derated spatial peak temporal average intensity (derated ISPTA) was measured 0.15 W/cm 2 .

The ND activation beam’s acoustic field was assessed through simulations using the Verasonics simulator and hydrophone measurements with the ONDA AIMS III system (Onda Corporation, CA, USA). The measurements employed a capsule hydrophone (HGL-0200, Onda Corporation, CA, USA) in degassed deionized water. The derated MI 0.5 at the activation beam’s spatial peak was measured.
ND detection algorithm
Each ND ultrasound image dataset contained 400 frames of baseline images and 400 frames of post-activation images ( N = 400). To isolate changes due to ND activation from baseline images, we performed frame-to-frame subtraction of the image at each pixel ( x, y ) to the first frame.
Equation (1) represents the difference between the post-activation frame and the baseline frame.
where I i n t e r p is the spatially interpolated version of I (interpolation factor = 4), and I ′ i n t e r p is the mean subtracted version of I i n t e r p ( Eq. 2 ).
To further improve spatial resolution and reduce uncorrelated spatiotemporal noise, the second-order cross-cumulant ( C second-order ) as introduced in super-resolution optical fluctuation imaging [ , ] was performed ( Eq. 3 ), which calculates the spatial coherence of adjacent pixels to preserve correlated signals (i.e., stationary extravasated NDs) and reject noise with low spatial coherence. As shown in Equation (3 ), a local window (3 × 3 pixels) was used for the spatial coherence measurements between pixels with 400 temporal samples, and the results were averaged temporally across these samples.
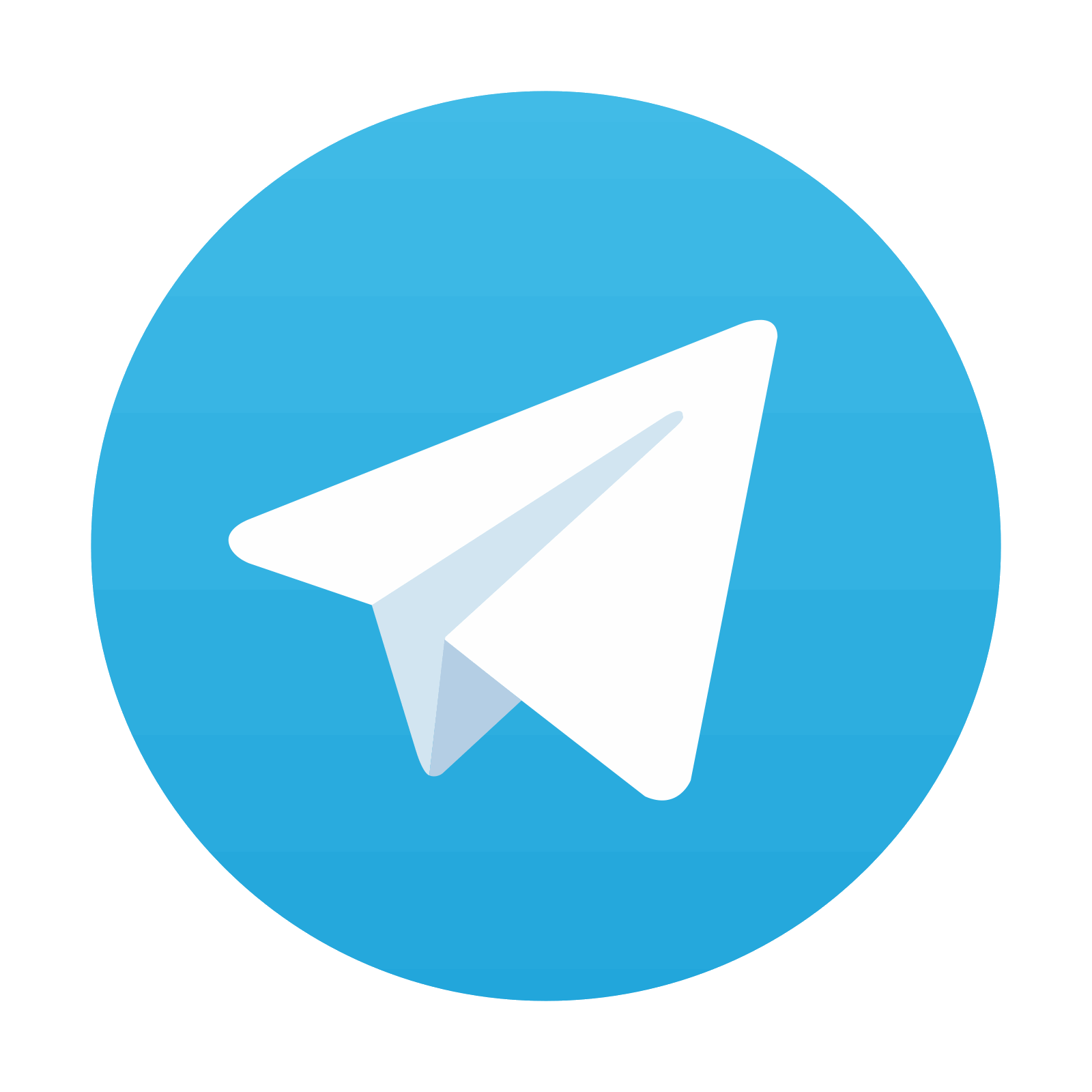
Stay updated, free articles. Join our Telegram channel
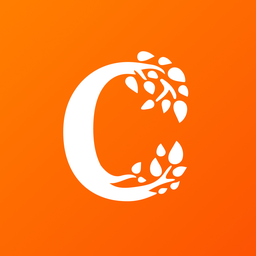
Full access? Get Clinical Tree
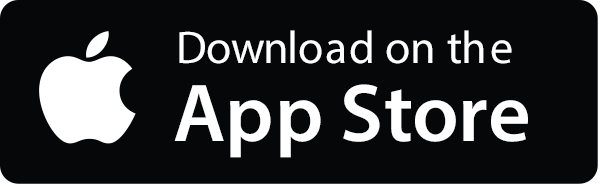
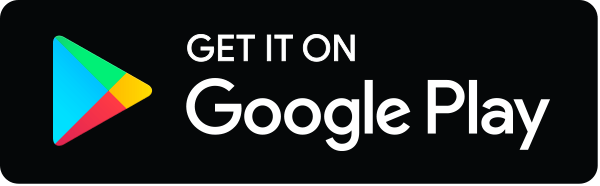
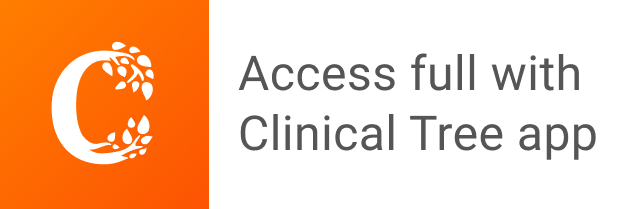