Traumatic brain injury (TBI) is a major health concern in the United States and worldwide. Neuroimaging is a critical element in the clinical evaluation of TBIs, as computed tomography (CT) and MR imaging are commonly used to identify structural changes that may aid in treatment decision-making and long-term patient monitoring. This article reviews the utility of CT and MR imaging while focusing on the emerging applications of PET in TBI. Pertinent research findings in the molecular imaging of cerebral metabolism, tau and β-amyloid, neurotransmitters, and neuroinflammation are discussed.
Key points
- •
Computed tomography (CT) is an appropriate neuroimaging method in acute traumatic brain injury (TBI) that is sensitive to pathology requiring immediate neurosurgical intervention but has limited prognostic value.
- •
Magnetic resonance (MR) imaging is commonly used in the subacute to chronic setting of TBI and is more sensitive than CT for the detection of brain abnormalities such as diffuse axonal injury.
- •
In both acute and chronic TBI, positron emission tomography (PET) reveals molecular changes that correlate with clinical outcomes such as persistent post-concussion syndrome and course of recovery.
- •
Multimodal imaging will enable a comprehensive assessment of TBI by coupling structural and molecular pathophysiology.
Introduction
Traumatic brain injury (TBI) is a leading cause of death and disability among children and adults in the United States. TBIs are classified as either a “bump”, “jolt”, or “penetrating” head injury that results in disruption of normal brain activity of function with 3 levels of grading/staging: mild, moderate, and severe. An average of 2 million Americans suffer from nonfatal TBIs annually with estimated healthcare costs exceeding 40 billion dollars for such injuries. TBI is responsible for 300,000 hospitalizations and 50,000 deaths in the United States each year, and even a singular TBI may increase patient mortality by 7-fold in the decade following injury.
The Glasgow Coma Scale (GCS) is a commonly used clinical scoring system that classifies TBI severity based on points associated with eye opening, motor response, and verbal response: mild (13 – 15), medium (9 – 12), or severe (3 – 8). , The GCS has faced criticism in the diagnosis of TBI as this score cannot provide any mechanistic or pathophysiologic information and may even be inaccurate due to the effects of sedation in the acute setting. TBI can be further classified into primary and secondary brain injury. Primary injury refers to brain damage directly upon impact that is often refractory to treatment. Such instances include external penetration of the skull, shearing forces resulting in diffuse axonal injury (DAI), and damage to blood vessels from compression and contusions. Secondary injury occurs after primary impact and includes cerebral edema, herniation, and altered cerebral flow such as ischemia or hemorrhage.
Neuroimaging is an essential step in the workup of TBI, providing an objective visualization of injury that supplements clinical diagnostic criteria and may provide prognostic insight into morbidity and mortality. This article will review the utility of neuroimaging in TBI, primarily focusing on the application of molecular imaging with PET while covering conventional imaging modalities such as X-ray computed tomography (CT) and MR imaging.
Computed tomography
Non-contrast head CT is considered to be the most appropriate initial neuroimaging workup in the setting of an acute TBI, particularly for moderate or severe TBIs (ie, GCS < 13). CT is a widely accessible imaging modality that can accurately and swiftly identify pathologies requiring prompt neurosurgical intervention such as acute hemorrhage, midline shift, or herniation. CT is also sensitive and specific for skull fractures and foreign bodies. In the event of an acute mild TBI, the decision to perform a CT is based on clinical scales such as the New Orleans Criteria, National Emergency X-Ray Utilization Study, or Canadian CT Head Rule. CT has been shown to have a negative predictive value of 99.7% for excluding significant intracerebral injury in patients with mild TBI, further validating the use of this modality in the acute setting.
Despite its value in triaging patients with acute TBI, non-contrast CT has several limitations that preclude its use in the subacute to chronic setting. Of most significance, CT cannot reliably detect certain patterns of brain injury such as DAI or secondary injury such as parenchymal contusions and edema. , As these pathologies principally drive negative long-term outcomes in patients with TBI, CT is considered a poor prognosticator of neuropsychologic and cognitive dysfunction.
Perfusion CT (PCT) is an advanced modality commonly used to evaluate cerebrovascular disorders such as an acute stroke by measuring cerebral blood flow (CBF), mean transit time (MTT) of blood through brain tissue, and cerebral blood volume (CBV). Assessing altered hemodynamics are also relevant for TBI, which can lead to ischemic changes such as edema and intracranial hypertension. PCT has been shown to be superior to non-contrast CT in the detection of perfusion defects associated with TBI, with a specificity of 93.9% for cerebral contusions. Contusions are characterized by decreased CBF, decreased CBV, and elevated MTT in focal cortical-subcortical regions detected by PCT ( Fig. 1 ). Similarly, intracranial hypertension can be identified by reduced CBF, reduced CBV, and increased MTT.
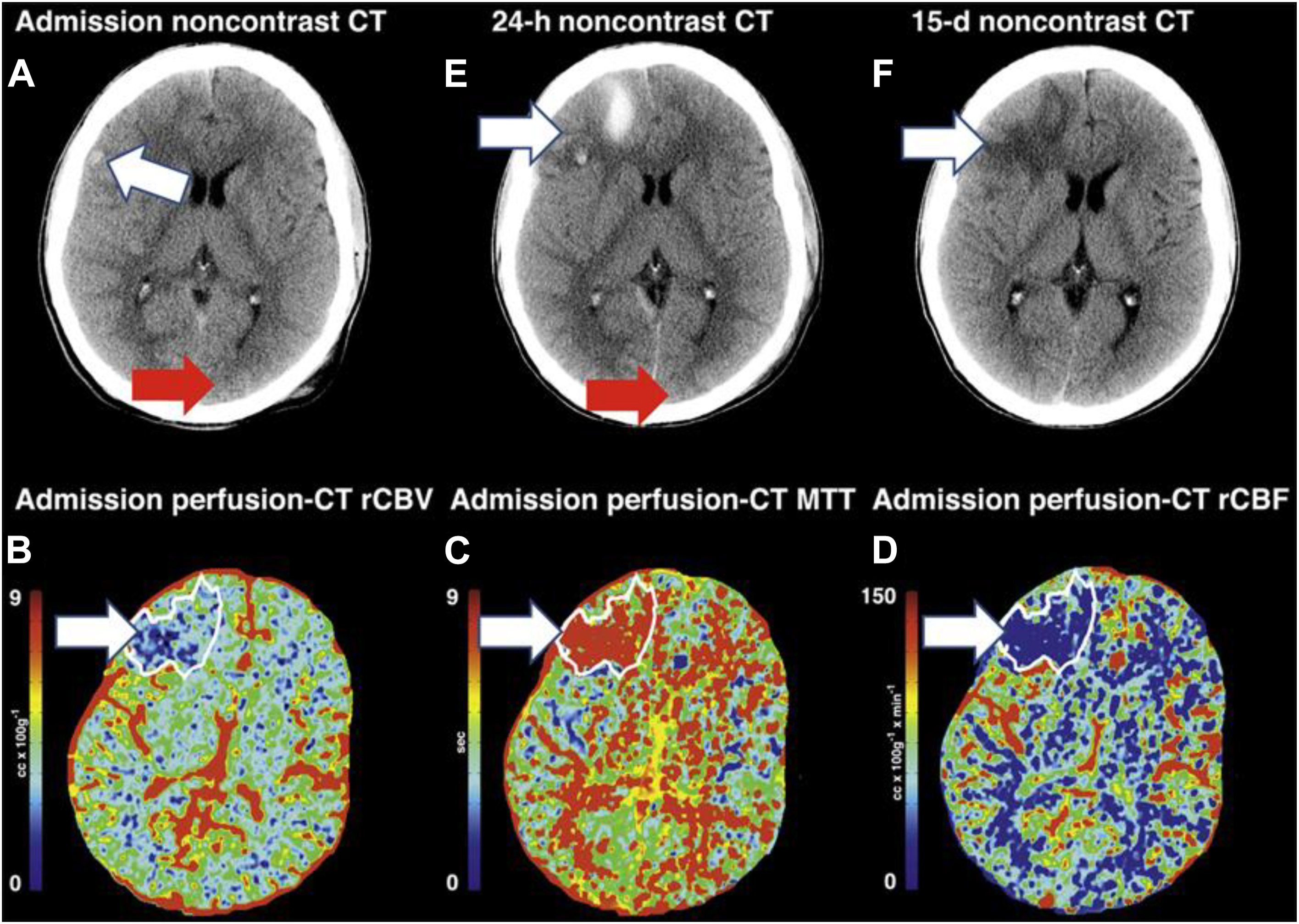
PCT can detect vascular injury even without visible defects on initial non-contrast CT and therefore may better correlate with long-term outcome. A study of 50 severe TBI patients found that PCT metrics obtained at initial evaluation had greater prognostic ability than pre-intubation GCS. An additional study by Metting and colleagues showed that reduced CBF and CBV in frontal and occipital regions were associated with poor functional outcome at 6 mo in 76 patients with mild TBI and unremarkable non-contrast CT.
MR imaging
Magnetic resonance (MR) imaging is particularly valuable in the subacute phase of TBI management. Conventional MR imaging is now almost universally accessible, enabling the practical use of this modality to image TBI. Studies have indicated that CT scans miss 10% to 20% of brain abnormalities detected by MR imaging in mild TBI ( Fig. 2 ). Between 48 and 72 ho post-injury, MR imaging outperforms CT in identifying swelling, bleeding, brain contusions, and axonal injuries. MR imaging is also considered to be more effective than CT at identifying DAI by providing detailed images of gray-white matter boundaries, which is crucial for volumetric comparisons.
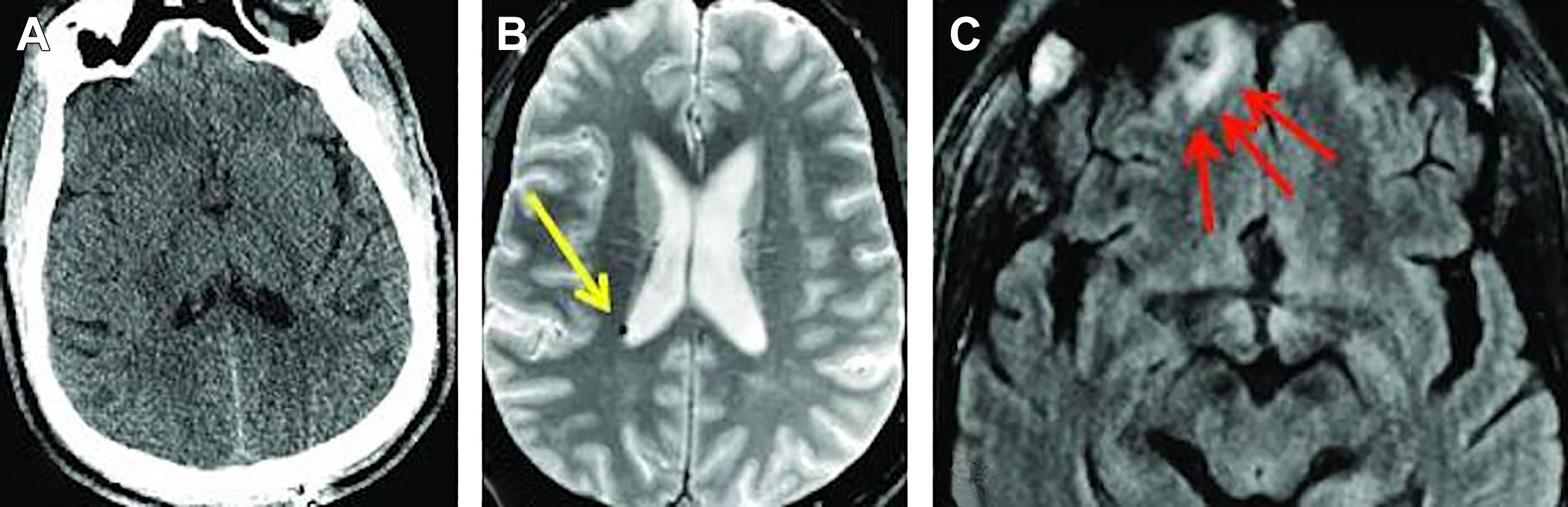
Fluid attenuated inverse recovery (FLAIR) is an MR imaging sequence that suppresses the high signal from cerebrospinal fluid, making it particularly useful in stroke settings and increasingly in TBI, where it helps predict severe injury outcomes. For instance, FLAIR imaging has shown promise in predicting outcomes for TBI patients with DAI. DAI can interrupt axonal transport and trigger neuroinflammation, abnormalities that can lead to neurodegeneration and persistent neuroinflammation predominantly in large white matter tracts.
Advanced MR imaging techniques such as magnetic resonance spectroscopy (MRS) and functional MR (fMR) imaging have additional applications in TBI. MRS is a technique that extends the capabilities of MR imaging by detecting magnetic field interactions between protons in a way that allows for quantification of chemicals in a given tissue. This allows for measuring the concentration of specific metabolites in the brain, which in turn provides a biochemical profile that reflects both metabolic health, as well as cellular changes such as neuron loss or demyelination.
Commonly measured metabolites from MRS include N-acetylaspartate (NAA), choline (Cho), creatine (Cr), myo-inositol (mI), and lactate. NAA is considered a marker of viable neurons, with reduction postulated to be an early indicator of brain injury. Cho reflects membrane turnover and inflammation, Cr indicates energy metabolism, mI is associated with glial activity, and lactate is a marker of anaerobic metabolism. The metabolic profile obtained through MRS can detect changes early after a TBI that are not visible on conventional MR imaging, making it a valuable tool for diagnosing mild TBI and DAI. Studies have shown that patients with mild TBI often exhibit reduced NAA levels, indicating neuronal loss or dysfunction, even when structural imaging appears normal. Decreased NAA/Cr ratios and elevated lactate levels have been associated with worse cognitive and functional outcomes. , Additionally, increased Cho/Cr and mI/Cr ratios suggest ongoing inflammation and gliosis, which are linked to poorer recovery.
MRS is further useful within the context of TBI as this modality also demonstrates an ability to track metabolic changes over time, providing a non-invasive means to monitor disease progression and response to treatment. For instance, changes in NAA levels can indicate neuronal recovery or ongoing damage, while alterations in other metabolites can reflect changes in inflammation or energy metabolism. , Studies of patients with TBI using MRS have demonstrated diffuse changes in Cho and/or NAA are associated with severity of injury, clinical symptoms, neuropsychologic function, and gross neurologic outcomes months after injury. Reduced NAA/Cho ratios in the basal ganglia of patients with chronic TBI have been related to motor scanning, psychomotor speed, and attention as assessed by neuropsychologic examinations.
Despite its potential in both acute and chronic contexts, the clinical application of MRS in TBI faces several challenges. The technique requires specialized equipment and expertise, particularly for multi-nuclear MRS, limiting its availability in routine clinical practice. Moreover, the interpretation of MRS data can be complex due to the overlapping spectral peaks of different metabolites and variations in metabolic changes across different brain regions and injury severities. This is only worsened by the lack of protocol standardization between MRS studies, including ROI placement, scan parameters, and magnet field strength. Such differences might limit the use of MRS as a quantitative biomarker in TBI.
fMR imaging is another modality that detects alterations in blood oxygenation and flow (typically the blood-oxygen-level-dependent or BOLD signal) that occur in response to neural activity. When a brain region is more active, its neurons consume more oxygen, and the local increase in blood flow leads to a relative increase in oxyhemoglobin and a decrease in deoxyhemoglobin, which can be detected as a changed ratio by fMR imaging. Applying fMR imaging in patients with TBI may reveal functional abnormalities that are not visible on conventional structural imaging. TBI often disrupts neural networks, and fMR imaging can identify these disruptions, providing a more comprehensive assessment of brain injury. Functional connectivity is deduced by the presence of correlated patterns of changes in blood flow across regions of the brain. Studies have shown that patients with mild TBI exhibit altered functional connectivity in several brain networks, including the default mode network, which is crucial for introspective and memory-related functions.
Despite its potential, fMR imaging has several limitations in the context of TBI. The technique is sensitive to motion artifacts, and results can be influenced by various factors such as age, sex, and comorbidities. Another challenge is the variability in study designs and methodologies, which makes it difficult to compare results across studies and draw definitive conclusions. Standardizing fMR imaging protocols and developing robust biomarkers are essential steps toward improving its clinical utility.
Diffusion Tensor Imaging (DTI) is a rapidly evolving MR imaging technique that detects white matter abnormalities across various neurologic conditions, including TBI. DTI visualizes water diffusion along white matter fibers, highlighting areas of altered diffusion indicative of axonal changes associated with TBI and DAI. DTI fractional anisotropy is a tissue organization index that ranges from 0 (isotropic) to 1 (anisotropic). Healthy neurons typically demonstrate anisotropic diffusion, during which water motion is restricted to the orientation of the axon. However, as the relative axonal membrane density and myelin sheath density decreases, diffusion becomes less anisotropic and thus mean diffusivity increases. As such, neuronal damage can be deduced by changes in fractional anisotropy. DTI studies on patients with TBI have frequently shown microstructural damage in white matter tracts of the corpus callosum, frontal lobe, internal capsule, and cingulum. , Reduction in white matter integrity as assessed by DTI has been shown to be inversely correlated with executive function and cognitive performance on tests of learning and memory.
PET
While not routinely performed in the clinical management of TBI, molecular imaging of head injury with PET is an active area of research. In contrast to structural imaging such as CT or MR imaging, PET may reveal insight into the biologically active processes occurring in the brain after injury. Binding of PET radiotracers in vivo can detect relevant pathophysiology such as changes in cerebral metabolism, accumulation of tau protein or beta amyloid, alterations in neurotransmitters, and neuroinflammation.
[18F] Fluorodeoxyglucose
[18F] fluorodeoxyglucose (FDG) is a widely used PET radiotracer that quantifies metabolic activity in the brain by acting as an analog of glucose. Following TBI, uptake of FDG may be impaired, which may manifest as hypo- or hyper-metabolism seen on a PET scan. Numerous studies have demonstrated significant changes in cerebral glucose metabolism after TBI, particularly in severe or moderate injury. These alterations can generally be characterized by an acute increase in glucose uptake followed by hypometabolism in the chronic setting of TBI and eventual recovery of stable metabolic activity ( Fig. 3 ). , In a study of 28 patients with severe TBI, Bergsneider and colleagues reported both elevated global and regional FDG uptake within 1 w of injury. A separate study showed that in the month following injury, patients with severe TBI have demonstrated a 42% average reduction in brain FDG uptake, with the strongest decreases found in the precuneus. Restoration of normal glucose metabolism is then considered to begin at 1 mo post-injury, though deficits may persist. It is important to note that findings reported in the literature exhibit a degree of heterogeneity, as studies have found different patterns of FDG uptake following TBI such as concurrent hypo- and hypermetabolism.
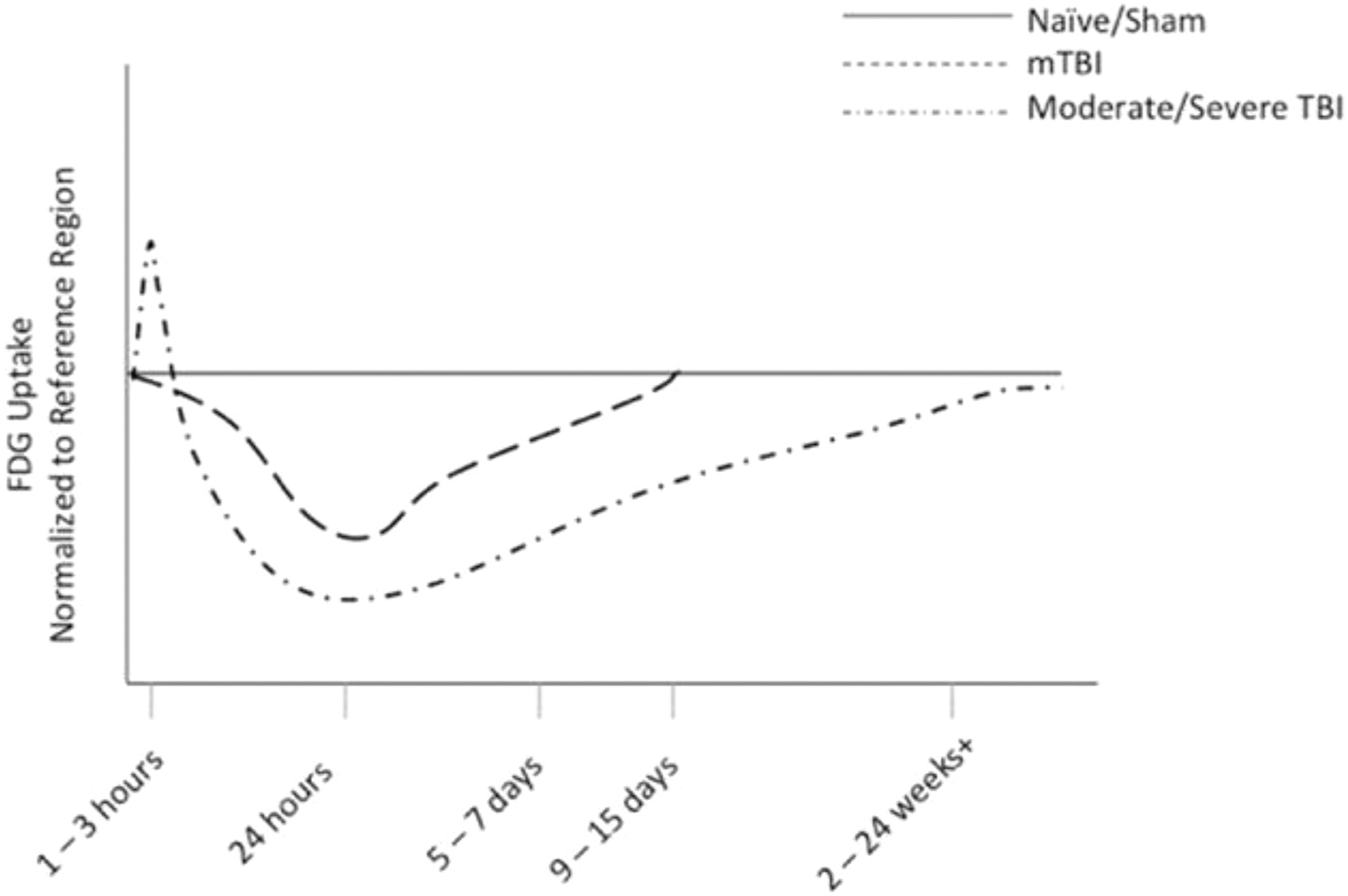
A limited number of studies have investigated the utility of FDG PET in mild TBI. Komura and colleagues identified patterns of both reduced and elevated FDG uptake in 89 mild TBI patients at 50 months post-injury: while hypometabolism was shown in the bilateral prefrontal region, hypermetabolism was observed around the limbic system. A separate study found decreased glucose metabolism in the parietal, somatosensory, and visual cortices of 34 military veterans with a 4-y history of blast-related mild TBIs relative to veterans without TBIs. FDG may also reveal the long-term impact of repetitive mild TBIs on cerebral function, demonstrating unique patterns of hypometabolism in patients with chronic traumatic encephalopathy (CTE) and frontotemporal lobar degeneration due to a history of repetitive TBIs ( Fig. 4 ).
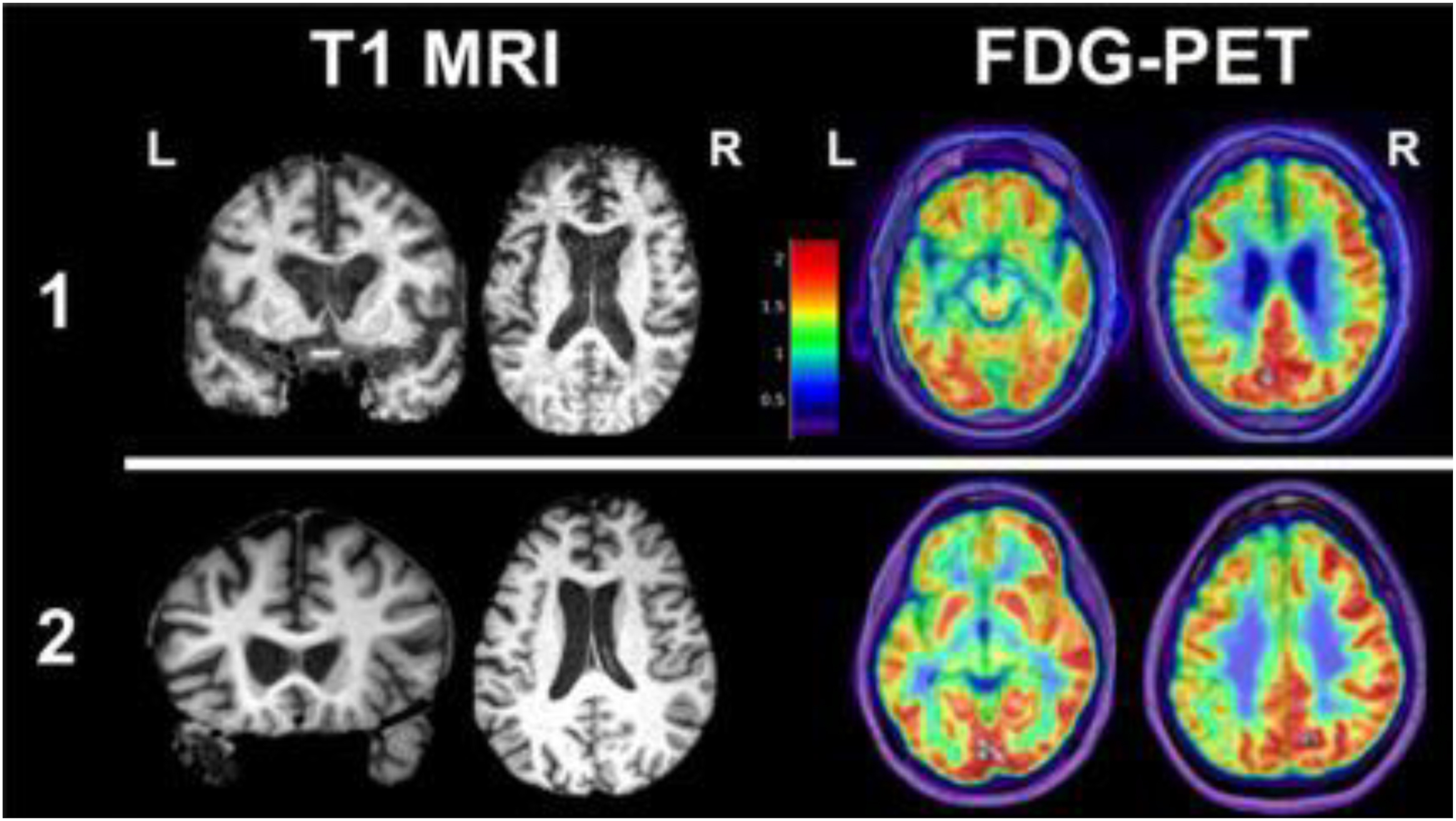
FDG PET studies have also revealed metabolic differences between diffuse and focal head injuries. Patients with focal cortical or extra-parenchymal injuries demonstrate reduced ipsilateral and contralateral cerebellar hypometabolism, a phenomenon termed crossed cerebellar diaschisis. Typically, the most severe focal lesions, as ranked by neurosurgeons, are predominantly associated with contralateral cerebellar hypometabolism. In contrast, patients with both focal and diffuse injuries do not exhibit a preference for laterality in cerebellar hypometabolism. Serial PET scans of diffuse injury have indicated that cerebellar hypometabolism is not consistent over time, possibly due to the reversibility of cerebellar diaschisis from acute brain injury or the competing effects of multiple lesions. In a study of 22 boxers, for whom focal trauma is more likely than diffuse injury, significant FDG reductions were observed in the cerebellum, as well as the posterior cingulate cortex, parieto-occipital lobe, and frontal lobe bilaterally.
Several studies have suggested that the metabolic changes visualized by FDG PET may correlate with long-term functional and cognitive outcomes of TBI, including post-concussive syndrome (PCS) and course of recovery. A study of 64 patients with persistent PCS demonstrated metabolic abnormalities in specific areas that support various cognitive processes, such as the anterior cingulate gyrus (correlating with cognitive changes) and the limbic structures (correlating with emotional changes). A separate study by Yamaki and colleagues conducted 2 FDG PET scans on 45 chronic mTBI patients about 2 y apart, identifying a number of patients (n = 22) with increased whole-brain metabolism and a group with stable FDG uptake (n = 23) at the second time point relative to initial imaging. The authors reported that patients with increased FDG uptake had better functional recovery, language and communicative ability, and wakefulness compared to patients with stable uptake.
Tau
Tau is a protein predominantly expressed in neurons that serves critical functions in microtubule-associated axonal transport and cytoskeleton stabilization. Disruption of cellular integrity can lead to hyperphosphorylation of tau and disassociation from microtubules, thereby promoting the accumulation of tau into cytotoxic neurofibrillary tangles (NFTs). Buildup of NFTs is associated with a number of neurodegenerative diseases collectively termed tauopathies, including Alzheimer’s disease, Parkinson’s disease, and CTE. CTE is a condition considered to arise from exposure to repetitive TBIs that induces diffuse deposition of NFTs, the presence of which confirms diagnosis on post-mortem histopathologic evaluation.
The potential utility that an in vivo biomarker of tau accumulation may provide in elucidating neurodegenerative pathology of TBI and CTE has driven efforts to develop numerous tau PET radiotracers. [18F] flortaucipir is one of the most prominent tau tracers that binds NFTs and has been used to assess tau aggregation in TBI and CTE. Stern and colleagues evaluated flortaucipir binding in 26 former National Football League (NFL) players and 31 healthy controls. Mean flortaucipir uptake was elevated in former players relative to controls in the bilateral superior frontal, bilateral medial temporal, and left parietal regions. Notably, the authors reported no association between tau uptake and performance on cognitive or neuropsychiatric tests. A recent analysis from the same group imaged a larger patient population (104 former NFL players, 58 former college football players, and 56 controls) and found significantly higher flortaucipir binding in the same regions among former football players compared to controls. Additionally, a correlation between flortaucipir uptake in the superior frontal region and exposure to repetitive head injury was reported in former football players above the age of 60.
A separate study by Lesman-Segev and colleagues assessed flortaucipir PET in 11 patients with a history of repetitive TBI and suspected CTE. Diffuse cortical tau PET uptake was observed in patients with significant binding of amyloid protein, while a pattern of fronto-temporal tau binding was found in amyloid-negative patients. Other studies have reported similar findings with different tau PET radiotracers in patients with repetitive sports-related concussions or TBIs ( Fig. 5 ). Importantly, tau radiotracers have been shown to exhibit significant off-target binding in the striatal, nigral, and gray-white matter interface. Non-specific uptake remains a notable limitation of flortaucipir and other novel tau radiotracers, which necessitates additional research initiatives to validate PET imaging of TBI-associated tauopathy.
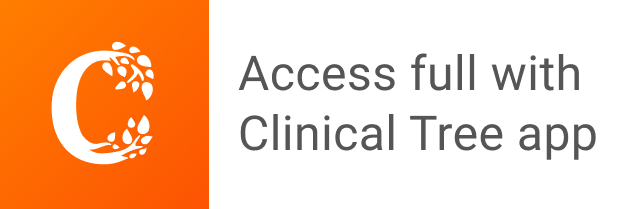