Abstract
Objective
Durable and stable phantoms for verifying and validating the new magnetomotive ultrasound technique are lacking. Here we propose a phantom design to address this need.
Methods
A mixture of styrene-butylene/ethylene-styrene (SEBS) in mineral oil and glass beads as a scattering material acted as a bulk material, in which a polyvinyl alcohol (PVA) inclusion containing magnetic nanoparticles in water solution and graphite was embedded. The design mimics nanoparticle-laden lymph nodes embedded in mesorectal fat, as would be the clinical scenario for diagnostic support of staging rectal cancer using magnetomotive ultrasound.
Results
The estimated reflection between the insert and bulk material was 10%, matching the clinical case of a lymph node within fat (9%). Speed of sound, attenuation, and Young’s modulus of the bulk material were matched with those of body fat. The insert also matched the acoustic and elastic properties of lymph node tissue except for attenuation, which was lower than that given in the literature. Glass beads and graphite were used to control backscatter levels in the respective tissue mimics, providing a contrast of -3.8 dB that was consistent with clinical image appearance. The magnitude of magnetomotion remained stable in three separate samples over the course of 3 weeks.
Conclusion
We have developed a phantom for magnetomotive ultrasound that combines the stability of an oil-based bulk material with the necessity of using a water-based material for the insert. The production procedure may be applied to other phantoms where one tissue type needs to be embedded within another.
Introduction
Magnetomotive ultrasound (MMUS) is an emerging novel technique that enables superparamagnetic iron oxide nanoparticles to be used as a contrast agent for ultrasound [ ]. A time-varying magnetic field acts to displace the nanoparticles, and as this motion is transferred to the surrounding tissue the resulting tissue vibration serves as a marker for the presence of nanoparticles.
This technique is presently employed within a medical device that is currently in the development phase. The goal of the device is to contribute to the health care system for rectal cancer diagnostics by providing more reliable detection of cancer spread to nearby lymph nodes, an important marker of cancer progression [ ]. Like all medical devices under development, verification and validation are essential to ensure optimal functionality and fulfillment with regulatory requirements. Animal and/or human studies are often necessary for evaluation of clinical inquiries, while more technical parameters are more efficiently tested using phantoms, which is often preferred for ethical, economical, and environmental reasons. As MMUS relies on ultrasound imaging, ultrasound tissue-mimicking phantoms [ , ] are the natural starting point for creating test objects. What sets an MMUS phantom apart is the inclusion of a magnetically labeled region, preferably using the same type of nanoparticles intended for clinical application.
Several additional requirements must be considered for a MMUS phantom, beyond accurately mimicking the acoustic properties of soft tissue. First, to ensure consistent behavior for repeatable measurements, the phantom material must be durable enough to withstand the aging processes, particularly those that could alter the properties of the nanoparticle compound. Moreover, acoustic parameters common to other ultrasound phantoms, such as sound speed, attenuation, and backscatter, must also remain stable.
Second, as contrast in a magnetomotive image is derived from nanoparticle motion, and this parameter is highly dependent on the viscoelastic properties, the capability to tune these is essential.
Third, for the specific case of rectal lymph nodes, these nodes are typically localized within the mesorectal fat, and in an ultrasound image, they may appear darker than the surrounding fat tissue [ ]. Thus, backscatter is another critical parameter to adjust to achieve this appearance.
Numerous materials have been suggested for the production of ultrasound tissue-mimicking phantoms, some of which are also used in MMUS research [ ]; for example, imaging [ ] and elastography [ ]. None of the MMUS phantoms presented have, however, been optimized for a specific anatomical situation.
In general, tissue-mimicking materials can be categorized into water-based or oil-based types, with water-based typically being less durable than oil-based. Styrene-butylene/ethylene-styrene (SEBS) in mineral oil mixed with ballistic gel offers the advantage of tunable elasticity without significantly affecting sound speed [ ]. However, nanoparticles for clinical use are dissolved in water and therefore do not mix with an oil-based matrix. This limitation necessitates the use of water-based materials such as gelatin [ ] or polyvinyl alcohol (PVA) [ ]. Notably, by varying the concentration of gelatin, the elasticity may be adjusted [ ]. For PVA, a water solution can be made to polymerize by the solution undergoing sequences of freeze-thaw cycles [ ], and its concentration in water as well as the number of freeze-thaw cycles determines the elasticity.
A drawback of water-based materials is that water tends to evaporate from the phantom, necessitating that it is completely sealed. Additionally, there is a risk of bacterial invasion, which may limit durability.
To meet the conflicting requirements of durability and water solubility, we propose the use of a bulk material consisting of an oil-based polymer (SEBS) with a sealed insert made from PVA containing the nanoparticles. This design mimics the anatomical scenario where magnetic nanoparticles accumulate in lymph nodes draining a tumor area after a peritumoral subcutaneous injection, following the proposed clinical strategy for MMUS.
To minimize reflection between the bulk material and the insert, here we suggested pouring the PVA solution into a designated cavity in the bulk material, followed by a freeze-thaw cycle of the entire volume. Sound speed and other acoustic parameters were adjusted to replicate the case of a rectal lymph node embedded in fatty tissue. Backscatter and attenuation were controlled by incorporating glass beads and graphite; graphite primarily regulates the desired attenuation, while glass beads adjust the backscatter. The stiffness of the bulk material was mainly determined by the concentration of SEBS to oil, whereas the stiffness of the insert depends on PVA concentration, along with the number of freeze-thaw cycles. Finally, the durability of the SEBS/PVA combination was tested over a 3 week period.
Methods
The aim of the phantom is to mimic a portion of the human rectum. However, as with all tissue phantoms, compromises were necessary to model this complex anatomical structure. Anatomically, the lymph nodes of interest are located a few millimeters to a maximum of 3 cm away from the rectal wall [ ] and are embedded in the mesorectal fat that surrounds the rectum. As the thickness of the rectal wall is only a few millimeters (2.14 ± 0.54 mm [ ]) and not the focus of this application, only the mesorectal fat with an embedded lymph node was modeled. This simplification may pose a limitation, as the rectal wall could introduce a phase-aberrating effect. However, as the rectal wall is stationary, it is less likely to affect magnetomotion estimation, similar to how the morphology of overlying tissues has a limited impact on blood flow velocity measurements. Depending on the extent of phase aberration, it could potentially degrade the focus, resulting in a blurrier image. Nevertheless, because the primary purpose of the phantom is to evaluate magnetomotion rather than image quality, phase-aberrating effects from the rectal wall were not considered in the phantom design.
Due to its complexity and small size, the internal structure of the lymph node itself was not modeled and an even distribution of nanoparticles within the node was assumed, as would be expected for healthy lymph nodes (metastases are visualized as particle-free voids within the node [ , ]). The two different parts of the phantom are henceforth referred to as the bulk material and the insert, mimicking the mesorectal fat and the lymph node, respectively. These should resemble the real tissue from an acoustic and mechanical point of view, and thus speed of sound, backscatter, attenuation coefficient, and elasticity were considered in the model.
Phantom construction
The manufacturing process for the bulk material and the insert, as well as their integration into a single unit, is outlined below. Each material has a section detailing general design requirements, followed by a section on the fabrication of each component.
Bulk—general design requirements
The bulk part of the phantom was made of SEBS-type copolymers mixed in mineral oil with added ultrasound scatterers. This specific copolymer was chosen for its tunable viscoelasticity and acoustic properties, which closely resemble those of human tissue. Notably, it offers temporal stability in its Young’s modulus as well as speed of sound and attenuation measurements similar to that of human fat [ ]. Additionally, SEBS phantoms are homogeneous, easy to produce, non-toxic, and relatively inexpensive. Composition of the phantom was initially based on the formulation proposed by Oudry et al. [ ], but the parameters were modified to more accurately match the conditions modeled in this study.
The aim was to have a Young’s modulus resembling human fat, measured by Samani et al. [ ] to be 3 ± 1 kPa. In polymerized SEBS, the Young’s modulus increases with concentration following the relation (Eqn [1]) given by Oudry et al.:
E=0.16c2.46
Attenuation is commonly adjusted in phantoms by adding graphite, but in SEBS it was found to cause excessive attenuation at the targeted frequency of 10 MHz. The attenuation coefficient for human breast fat was found by d’Astous and Foster [ ] to obey the relation 0.158 ± 0.03 dB/(cm MHz 1.7 ), valid between 3 and 7 MHz. However, a wide range of values with large uncertainty has been reported for human fat, even up to several dB cm −1 MHz −1 [ , ]. Based on observations from our ongoing clinical study, we chose to evaluate the relation by d’Astous and Foster at 10 MHz. This calculation resulted in a value of 7.9 dB/cm, a value more closely achieved by adding glass beads only at a concentration of 3 wt%.
The speed of sound in mesenteric fat was found by Errabolu et al. [ ] to be 1427 m s −1 , while for fat tissues in general it can reach up to 1470 m s −1 [ , ].
Bulk material preparation
The bulk material was prepared in an autoclave bottle. In total, 94 g of mineral oil with a dynamic viscosity of 14.2–17 mm 2 s −1 at 40°C (catalog no. 330779, Sigma-Aldrich, Darmstadt, Germany) and 3 g of SEBS (Kraton G 1650E, Kraton Polymers, Houston TX, USA) were mixed before being heated in a temperature-controlled oven (VWR DRY-Line, VWR, Radnor PA, USA) at 120°C for 2 h. A stirrer magnet was placed inside the bottle prior to heating to prevent the formation of gas bubbles when placed on the hot plate. At this step, only 90% of the final mineral oil mass was used. The remaining 10% was reserved to pre-mix the glass bead powder (type A2429 glass, Potters Industries, Malvern, PA, USA) before adding it to the main solution. This approach helped to prevent glass bead aggregates, maintaining a concentration 3 wt% of the total solution. Once this secondary solution was homogeneously mixed, it was added to the heated SEBS solution and stirred at 500 rpm for 5 min. This speed was chosen as a balance between preventing bead sedimentation and ensuring an even distribution of heat throughout the solution.
After mixing, the mixture was slowly and carefully poured into the mold ( Fig. 1 ) and allowed to cool at room temperature, during which the gel formed. The temperature of the solution during pouring was critical; if too low, it may trap bubbles that form while pouring the viscous liquid. Conversely, if poured when too hot, the glass beads may have time to sediment before the solution solidifies. A total of 20 mL of the bulk solution was reserved and kept at room temperature to be used as a sealant at a later stage. Note that from this point, the gel could be re-used by re-heating it.
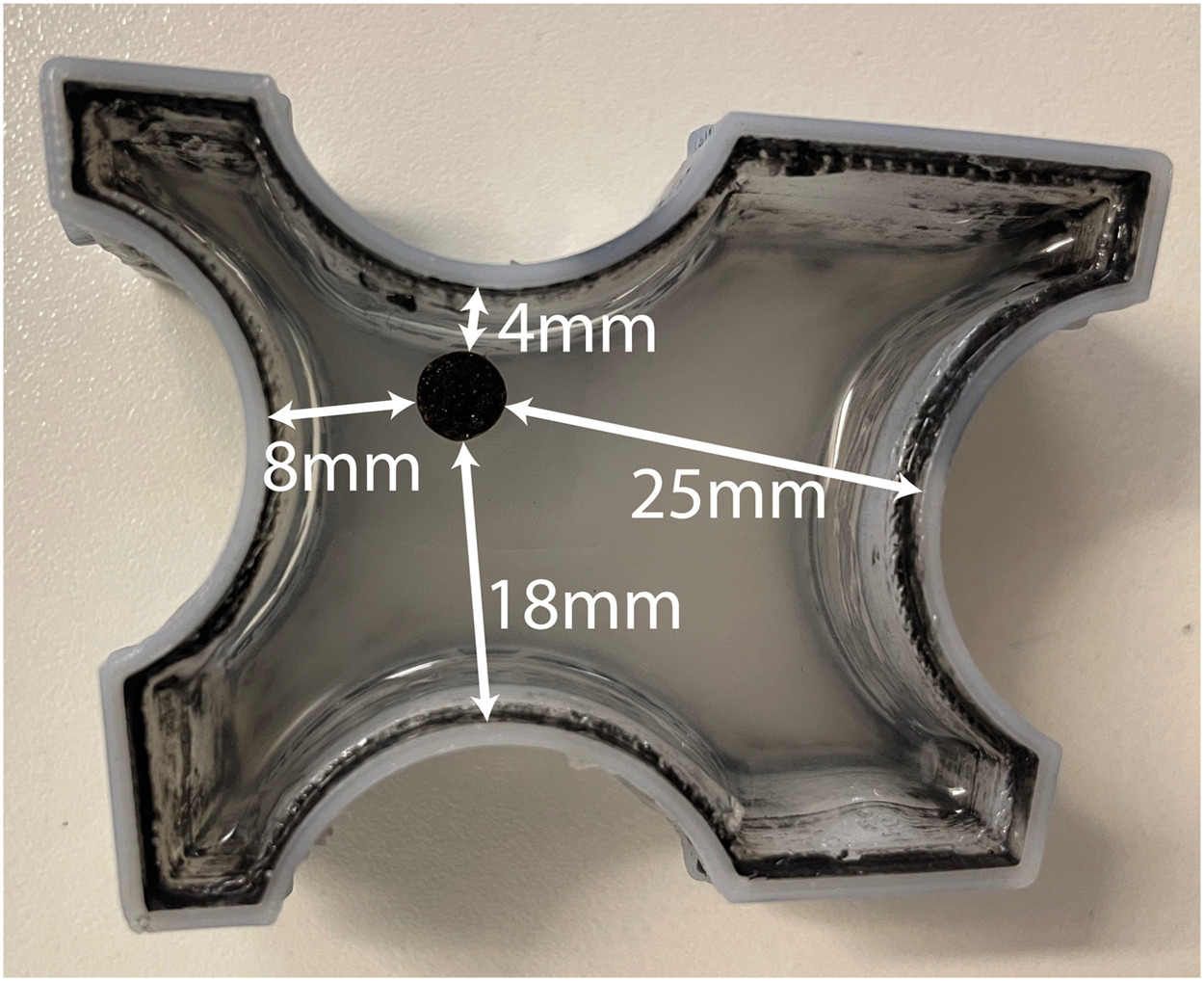
Solutions with glass bead concentrations of 0, 1, and 2 wt% were prepared similarly.
Insert—general design requirements
The nanoparticles used were water-soluble, necessitating a water-based phantom material. For this reason, a PVA polymer was chosen as the material for the insert. As the insert was designed to be encapsulated in the bulk material, we hypothesized that water evaporation from the PVA should not be an issue for the phantom’s durability. The production method of the insert was inspired by Surry et al. [ ]. However, the parameters were adjusted to achieve an acceptable balance between viscoelastic and acoustic properties to better resemble a human lymph node.
For modeling magnetomotion, the principal parameter for the insert was Young’s modulus, which should reflect the elastic properties of a lymph node. For axillary lymph nodes, this was measured at 6.9 ± 0.5 kPa [ ]. In a PVA phantom, elasticity depends on PVA concentration as well as the number of freeze-thaw cycles applied during fabrication. Several studies have suggested different PVA concentrations and freeze-thaw cycles to simulate biological tissue [ ]. Three PVA concentrations of 10, 12.5, and 15 wt% at a single freeze-thaw cycle were evaluated under these conditions based on Young’s modulus reported by Gordon [ ] to find the best match for the aforementioned lymph nodes.
Sound attenuation in human lymph nodes has been measured as 0.58 dB cm −1 MHz −1 [ ]; however, unclear over over what frequency range. As lymph nodes appear darker than their surrounding in an ultrasound image, graphite was preferred to achieve this appearance because it produces less scattering than glass beads. Additionally, graphite is able to increase attenuation to levels similar to those of lymph nodes. Note, however, that it was not possible to reach the desired attenuation by adding more graphite, as this would have increased echogenicity too much. Concentrations of 5, 15, and 30 mg mL −1 were investigated for sound speed, attenuation, and Young’s modulus, as well as apparent backscatter relative to the bulk tissue.
Sound speed in human lymph nodes was found by the same investigators to be 1514 m s − 1 [ ].
Insert preparation
The material for the three inserts was made from PVA powder (Molecular weight 89,000–98,000, 99+% hydrolyzed, product no. 341584, Sigma-Aldrich, Darmstadt, Germany) at mass fractions of 10, 12.5, and 15 wt%. These were mixed with water to a total weight of 100 g for each sample and then heated in the oven at 100°C for 1 h. Water evaporation was compensated by adding water to maintain the correct PVA concentration. After heating, graphite powder was added at 5, 15, and 30 mg mL −1 for the 15 wt% PVA solution and mixed with a magnetic stirrer for 5 min at 500 rpm. The nanoparticles (Ferumoxtran-10, Ferrotran SPL Medical B.V., Nijmegen, The Netherlands) were prepared at different concentrations of 0.5, 1, 2, and 4 mg mL −1 and then directly added to the PVA and graphite solution. The preparation was left at room temperature to allow air bubbles to rise to the surface, which occurred spontaneously due to the solution’s low viscosity.
Solidification and polymerization of the PVA was induced by one freeze-thaw cycle of the entire bulk-insert combination (see the Measurements section below for phantom properties/Young’s modulus).
Bulk and insert combination—general design requirements
Due to the different properties of the base materials, it was found that the best way to avoid trapping air between them was to pour the insert in its molten form directly into a suitable cavity within the bulk material. Even so, the difference in acoustic impedance between the two substances should be similar to that observed in vivo . Fat, lymph nodes, and water have an acoustic impedance of 1.33 × 10 6 , 1.6 × 10 6 , and 1.48 × 10 6 kg m −2 s −1 , respectively [ ], while mineral oil has an acoustic impedance of 1.21 × 10 6 kg m −2 s −1 [ ]. Calculating the reflection coefficient for the two cases (with the PVA solution assigned the acoustic impedance of water) showed that the estimated reflection in the phantom was 10% compared with 9% in the tissue case. This calculation also neglects the influence of scattering from the presence of graphite and glass beads, or cellular structures in tissue.
The geometry of the final phantom was designed to allow imaging of the nanoparticle-laden insert from four directions by placing the transducer in semi-circular cutouts on each side of the insert ( Fig. 1 ). The insert was positioned to reflect the range of depths at which lymph nodes are typically found in humans [ ]. The height of the phantom was set at 10 mm, significantly greater than the elevation of the ultrasound image slice, providing some margin for placement of the transducer, including tilt.
Bulk and insert preparation
The mold for the bulk material was designed with a solid rod-shaped negative mass measuring 5 mm in diameter and 10 mm in height. After the gel had solidified and the rod was removed, the PVA solution could be poured into the hole left by the rod.
Before undergoing the freeze-thaw cycle, the insert solution was poured into the hole left in the bulk with the help of a syringe, which was tilted to minimize air bubbles. The bulk and insert ensemble were then placed in the freezer, with a single freeze-thaw cycle consisting of 18 h in the freezer at -20 ± 2°C followed by 2 h at room temperature.
After the freeze-thaw cycle, the remaining SEBS solution was reheated in the oven at 100 ± 5°C for 30 min and then poured over the hole containing the PVA insert, ensuring it was entirely enclosed by SEBS and thereby preventing exposure to air.
Measurement of phantom properties
Young’s modulus
The Young’s modulus of both material components of the phantom, the bulk material and the insert, was measured individually using separately molded 75 × 35 × 35 mm blocks prepared as described for each material (the PVA also underwent one 18 h freeze-thaw cycle). The material samples were positioned on a weight scale and compressed using a translation stage. This setup allowed measurement of both the applied force and the change in length, which were then used to calculate stress and strain.
For the bulk material, stress and strain were plotted and the Young’s modulus was obtained from linear regression between the two variables. The PVA samples exhibited a more non-linear behavior between stress and strain, so the mean static Young’s modulus value was determined as the slope of this relationship in a strain range between 3% and 8%, as done by Duboeuf et al. [ ].
Speed of sound
The sound speed in the SEBS bulk material samples was measured using a 2 MHz transducer driven by a pulser receiver (Panametrics 5052PR, Olympus NDT Inc, Waltham, MA, USA) set in pulse-echo mode. The phantom was placed in a water tank, and echoes from the front and back walls were recorded on an oscilloscope. Sound speed was determined by measuring both the length of the phantom sample (multiplied by two) and the time elapsed between the echoes.
The high attenuation made pulse-echo measurement challenging for the PVA insert samples, so the through-transmission technique described by Chen et al. [ ] was used instead. During the measurements the temperature was 19°C, which was used to calculate the sound speed in water according to Lubbers and Graaff [ ].
Measuring the length of the PVA samples proved difficult due to the softness of the material and the uneven surface left open to transmit sound. As a result, the length measurement had relatively low accuracy (75 ± 0.5 mm), leading to higher uncertainty in sound speed measurements.
Attenuation coefficient
The attenuation coefficient of each part of the phantom was measured by transmitting ultrasound pulses through the rectangular samples (75 × 35 mm) along their long and short axes, respectively. A 10 MHz transducer (Olympus A327S-SU, Olympus NDT Inc.) was placed coaxially in front of a 0.5 mm hydrophone (Precision Acoustics, Higher Bockhampton, Dorchester, UK) in a water tank, with the sample positioned between them. The transducer was driven by a pulser/receiver (Panametrics 5052PR, Olympus NDT Inc.). After fast Fourier transform calculations of the received signals, the power spectra (expressed in dB) from the two cases were subtracted, yielding the power lost over the length corresponding to the difference between the length and the width (40 mm) for each sample, for each frequency component within the usable bandwidth for the received signals. The attenuation emanating from the added water path when measuring the short axis was much less than the expected measurement errors and was not accounted for. This method minimized errors caused by reflections occurring at the sample interfaces.
Magnetomotive displacement measurements
A MMUS imaging system from NanoEcho AB (Lund, Sweden) was used to measure material displacement induced by the magnetic field generated by the system. This system is described by Jansson et al. [ ], but in a version that is now updated with a center frequency of the transducer elements of 10 MHz. Displacement in the samples for each nanoparticle concentration (0.5, 1, 2, and 4 mg mL −1 at a PVA concentration of 14%) was calculated as the average displacement recorded within a circular region of interest, drawn slightly smaller than the insert in the ultrasound image (diameter of 4.5 mm). The final displacement value was determined as the average of three individual measurements taken at different image planes. The limited thickness of the phantom allowed for only three independent planes—at the center, near the top, and near the bottom—facilitating repeatable measurements.
Assessment of durability
Three additional phantoms were produced according to the procedure outlined above and stored at room temperature. Over a period of 3 weeks, the weight and magnetomotive displacement of each phantom were recorded nearly every other day. The regions of interest were selected as previously described, and the distance in the ultrasound image from the transducer face to the center of the insert was noted for each measurement.
Results
Bulk
Young’s modulus
The Young’s modulus for the bulk was found to be 2.1 ± 0.5 kPa for a copolymer concentration of 3 wt%, as shown by the graph equation in Figure 2 (R 2 = 0.99). The theoretical values computed according to eqn (1) predict a Young’s modulus of 2.38 kPa.
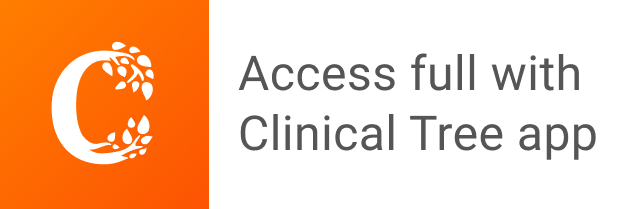