Abstract
Here we propose an ultrasound contrast-based imaging method that enables non-invasive quantitative assessment of ambient pressure changes inside the body (such as blood pressure). We subject the microbubbles in the contrast agent to two frequencies: A low-frequency (57 kHz) signal that dynamically manipulates the ambient pressure, and a series of high-frequency (4 MHz) pulses for exciting and imaging the bubble response. The imaging pulses exploit the ambient pressure sensitivity of the subharmonic microbubble response, while the low-frequency signal provided an intrinsic calibration for measurement of ambient pressure changes. We tested this approach in an in vitro setup and show that it can visualize and quantify ambient pressure differences with a sensitivity of 0.5 dB/kPa.
Introduction
Blood pressure is an important indicator for cardiovascular health, and interstitial fluid pressure is important in cancer diagnosis [ ]. The intraluminal pressures inside the different heart chambers and blood vessels are of high importance for assessment of heart function, severity of vascular stenoses, and loading conditions of the heart [ ], However, such intraluminal pressures can currently only be measured by invasive means such as catheters and pressure wires, and non-invasive means of measuring these pressures would be highly welcomed, particularly for use as early-stage biomarkers. Ultrasound contrast agents, specifically microbubbles, have been explored for many years as potential non-invasive pressure sensors as the acoustic behavior of the microbubbles is known to be highly dependent on the surrounding fluid pressure.
In contrast-enhanced ultrasound (CEUS), lipid-coated gas-filled microbubbles (diameter 1–10 μm) are administered intravenously and imaged with low acoustic amplitudes. Due to the non-linear properties of the viscoelastic microbubble shell, the effective stiffness and effective resonance of the microbubbles changes drastically with small size changes [ , ], such as those induced by a small ambient pressure difference. The maximum subharmonic scattering – which occurs when the resonance of the bubble matches half the ultrasound driving frequency – changes strongly with ambient pressure [ ].
However, the mechanics of coated microbubbles, and hence the subharmonic scattered amplitude, also depend on other factors than ambient pressure. For example, the subharmonic scattering changes over time through dissolution [ , ], and subharmonics are enhanced by the presence of iodinated contrast [ ]. Subharmonic sensitivity to ambient pressure, i.e. , the slope of the subharmonic as a function of static over-pressure, is also strongly influenced by the acoustic frequency and amplitude [ ]. Consequently, the values reported for subharmonic sensitivity to ambient pressure differs substantially across studies, even for well-controlled in vitro measurements [ , ].
Despite these dependencies on external factors, the clinical value of non-invasive pressure estimation via the subharmonic scattered intensity in CEUS imaging has been demonstrated repeatedly in vivo [ ]. The subharmonic amplitude has been shown to be particularly sensitive to small pressure changes, more so than scattering at other harmonics [ , ]. SubHarmonic Aided Pressure Estimation (SHAPE) [ ] has been investigated clinically for its potential to predict the therapeutic response of breast lesions to chemotherapy [ ], and for its potential in diagnosing portal hypertension [ ]. The subharmonic scattering values over a cardiac cycle also correlate with blood pressure catheter readings [ ], which potentially allows qualitative monitoring of variations in pulse pressure. However, the sensitivity of subharmonics to ambient pressure also depends on the pulse shape [ ], which suggests that calibration values are uniquely tied to the used ultrasound equipment. Furthermore these calibration values, which are obtained via in vitro scattering measurements as a function of static over-pressure, can underestimate the in vivo sensitivity [ ]. To overcome the limitation of unknown acoustic pressure in vivo , SHAPE studies now make use of an optimization scheme that determines the optimal acoustic pressure by maximizing the sensitivity of the subharmonic intensity to the acoustic pressure, and by extension to the ambient pressure [ ].
A more robust calibration procedure could allow quantitative non-invasive pressure measurements via subharmonic scattering with great diagnostic value for oncology and cardiovascular disease. The approach we propose here is to apply a low-frequency (kHz range) acoustic wave as a dynamic manipulation of the ambient pressure, similar to what was employed by Faez et al. [ ]. Performing regular pulse echo imaging over a dynamically manipulated ambient pressure could, in principle, measure the subharmonic sensitivity to ambient pressure, as shown in the scattering experiment by Faez et al. [ ]. In that in vitro setup, separate single-element transducers from different angles were used, but this could in principle also be done clinically using a dual-frequency transducer. While dual-frequency CEUS imaging has been used to enhance the contrast-to-tissue ratio [ ], here we instead test whether it can provide real-time feedback on the subharmonic sensitivity to ambient pressure [ ].
Methods
In this section we describe two different setups employing this dual-frequency approach that we use to characterize the method and validate the results: one that uses cross-propagation of the kHz wave and the imaging waves, and another using co-propagation. The cross-propagating setup directly images dynamically manipulated subharmonic scattering and is also used to optimize for microbubble concentration and acoustic amplitude. The optimized settings are then used in the co-propagating setup, which is closer to the intended imaging implementation, described later, and the results are compared with an externally applied static ambient pressure (SAP).
Cross-propagating setup
The cross-propagating setup consisted of a large water tank around a smaller 800 mL tank that contained the microbubble suspension ( i.e. , the bubble tank), shown schematically in Figure 1 . The open-top bubble tank was continuously stirred magnetically and filled with room-temperature water (left overnight prior to the experiment) and with SonoVue (Bracco Suisse SA, Plan-les-Ouates, Switzerland) microbubbles, of which the filling gas was substituted by C 4 F 10 gas, following the instructions of Kanbar et al. [ ]. The distance between the bubble tank and the wall of the water tank was approximately 8 cm. The bubble tank has two acoustic windows (polymethylpentene sheets of 2 mm thickness, see dotted lines in Fig. 1 ) from which the low-frequency acoustic waves could enter and exit. Because the acoustic impedance of these windows was close to that of water (1.44 [ ] and 1.48 MRayl [ ], respectively), reflections were negligible.
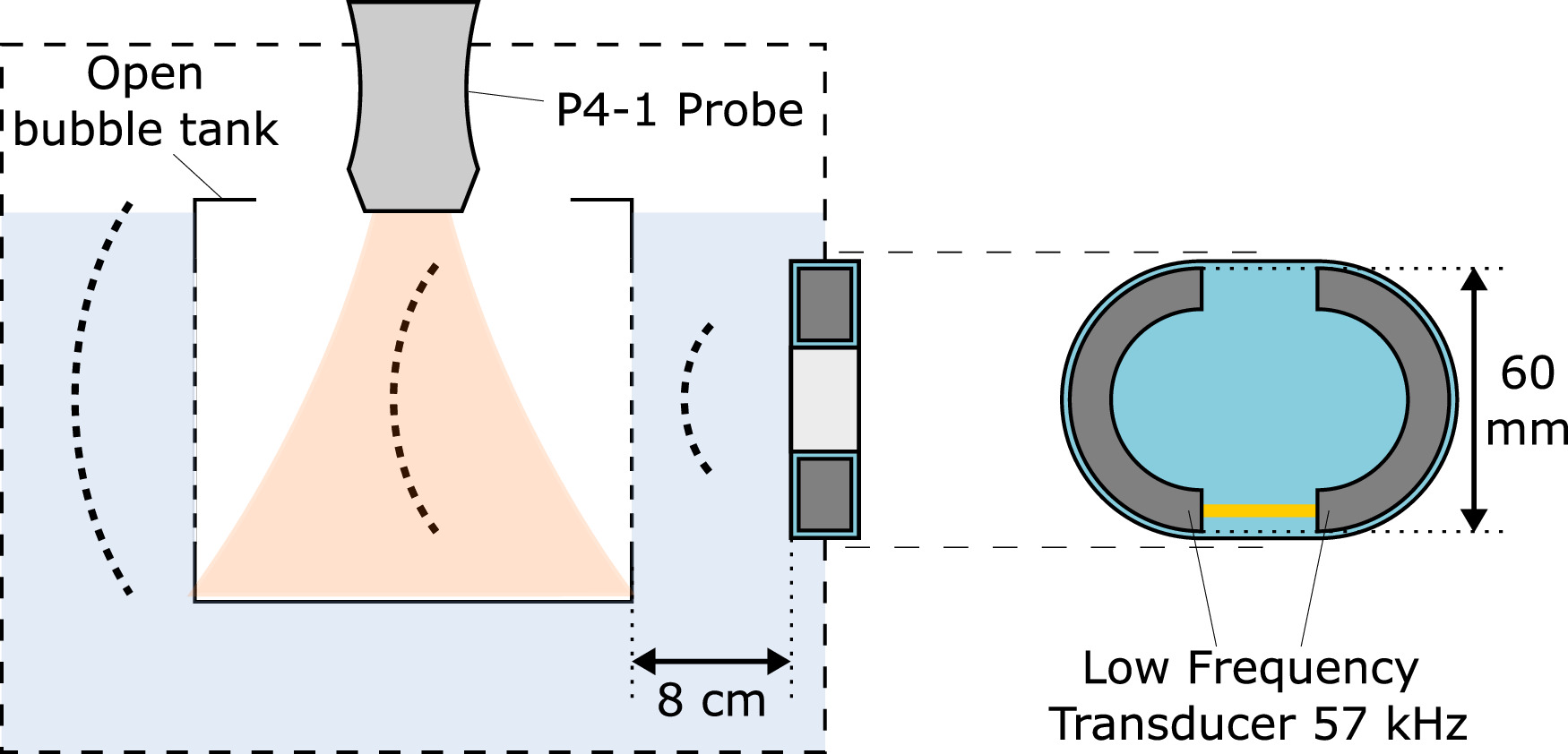
The dynamic ambient pressure (DAP) was induced by a ring-shaped low-frequency transducer (DH-43 60 × 30 × 10 mm, P4P8 piezoelectric ceramic ring, Shenzhen More-Suns Electronics Co., Ltd., Shenzhen, China). This low-frequency single-element transducer was cut in half, then reconnected electrically and sealed inside a custom holder to allow co-propagation with the imaging probe, see Figure 3 . This transducer generated a 57 kHz, 10 cycle pressure wave Gaussian tapered over the first two and last two cycles. The acoustic field of this low-frequency wave was characterized with a hydrophone (TC4038, Teledyne RESON, Slangerup, Denmark) and found to be quite non-homogeneous, with peak-to-peak acoustic pressures ranging from 2 to 10 kPa (15 to 75 mm Hg) in the region of the bubble tank.
The imaging probe (ATL P4-1, Philips Healthcare, The Netherlands) was coupled to a fully programmable Vantage 256 ultrasound system (Verasonics Inc., Kirkland, WA, USA), and its high-frequency transmit burst was delayed by 105 μs with respect to the DAP wave by an external trigger signal to allow the low-frequency wave to reach full amplitude at the imaged location. The imaging probe transmits a 4 MHz, eight-cycle Gaussian-tapered, diverging wave with a pulse repetition frequency (PRF) of 100 Hz. The DAP amplitude was set to 0 for 16 frames after each 16 th frame to obtain an equal number of modulated frames and unmodulated frames as a reference, representing the SAP.
Optimizing bubble concentration and acoustic pressure
The subharmonic amplitude was measured while varying the acoustic amplitude and testing the following bubble concentrations: 25, 50, 100, 200 and 400 μL to 800 mL (1 × 10 4 , 2 × 10 4 , 4 × 10 4 , 8 × 10 4 , 16 × 10 4 microbubbles/mL). For each concentration, the peak acoustic amplitude was swept from 14 to 70 kPa (28–140 kPa peak to peak) in 1.75 kPa steps, and 1280 frames (640 modulated and 640 reference) were recorded at each setting. Recording, storing and subsequently starting the new sequence took approximately 30 s. After this measurement sequence, another measurement sequence was performed on the same microbubble suspension to confirm the microbubble stability. In this second measurement, the pressure was increased from 14 to 26 kPa, as this is where sensitivity was most visible in preliminary measurements, with the same 1.75 kPa step size used. The microbubble suspension was refreshed before each concentration.
Image processing
The recorded radio-frequency data were beamformed once with the full bandwidth, and once with a filter around the subharmonic frequency (1.8–2.2 MHz). The 5 × 32 × 1280 beamformed images were grouped and averaged, such that for each acoustic amplitude and bubble concentration four images were obtained: one unfiltered without DAP ( Fig. 2 a), one unfiltered with DAP ( Fig. 2 b), one filtered around the subharmonic without DAP ( Fig. 2 c), and one filtered around the subharmonic with DAP ( Fig. 2 d). The diagonal stripes in the modulated subharmonic image ( Fig. 2 d) are a direct manifestation of the microbubbles’ subharmonic sensitivity to the DAP, which was not visible in the fundamental signal nor in the reference images, see Figures 2 a–c. These stripes are diagonal because the low-frequency wave traverses the imaging plane in a lateral direction at the same speed of sound as the imaging wave traverses it axially [ ]. The subharmonic intensity variation in axial direction was extracted in the frequency domain to find the optimal acoustic imaging amplitude and microbubble concentration. Note that this subharmonic intensity variation (dB), in principle, could be used as a measure of the sensitivity (dB/kPa). However, as the applied DAP amplitude varied considerably over the imaged area, and, also considering that the intensity variation from the DAP was not compared with a SAP change in this experiment, the exact subharmonic intensity variation was not considered a meaningful measure for the sensitivity to ambient pressure. One should also note that because of noise, the change in subharmonic intensity did not go down to 0 dB completely.
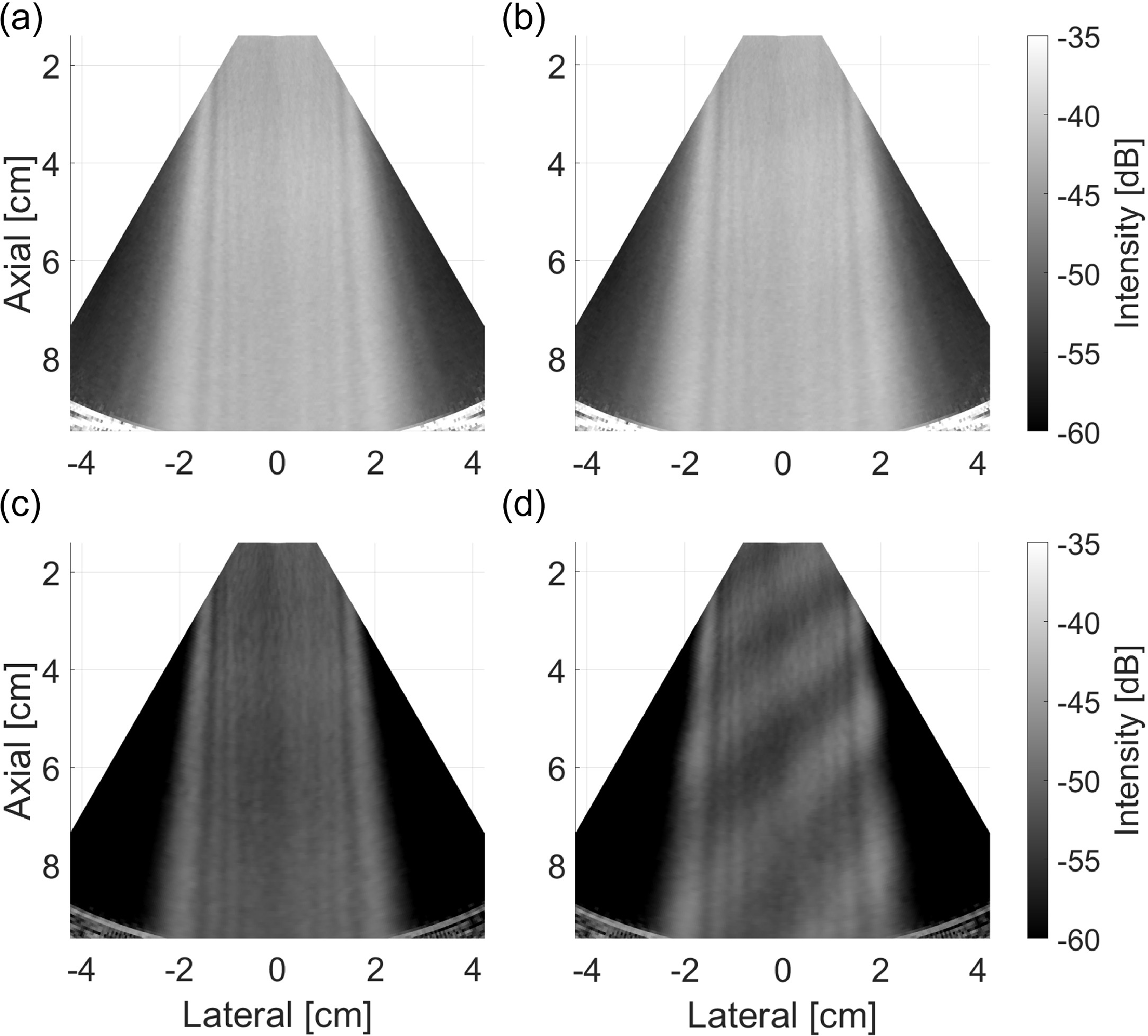
Co-propagation setup
This setup was used to test whether the sensitivity measured from the DAP manipulation matched the measured sensitivity in case of a change in SAP ( Fig. 3 ). It consisted of the same large water tank around a sealed bubble tank that contained the room-temperature bubble suspension and a slice of tissue-mimicking material. This bubble tank can be pressurized with a cuff pump equipped with a manometer (Heine Gamma G5; Heine Optotechnik, Herrsching am Ammersee, Germany). The applied pressures from the cuff pump were confirmed separately with a Delta-Cal (Utah Medical Products, Salt Lake City, UT, USA). The closest wall of the bubble tank was placed approximately 9 cm from the dual-frequency transducer, which was mounted on an acoustic window on the outside of the large water tank. This dual-frequency transducer consists of a low-frequency transducer and a P4-1 imaging probe, both placed inside the custom holder. In this manner the low-frequency wave and imaging wave co-propagate, with the resulting pressure in the image, i.e. , the instantaneous ambient pressure, the sum of the SAP and the DAP [ ]. By varying the phase offset of the DAP wave with respect to the imaging pulse, the resulting instantaneous ambient pressure can be varied per image. As the DAP field was not homogeneous over the entire imaged frame, a smaller region of interest (ROI; 1.5 × 1 cm) at a depth of 130 mm, as indicated in Figure 3 , was selected. The DAP amplitude in this ROI was 5.4 ± 0.4 kPa peak to peak (corresponding to 40 ± 2.9 mm Hg).
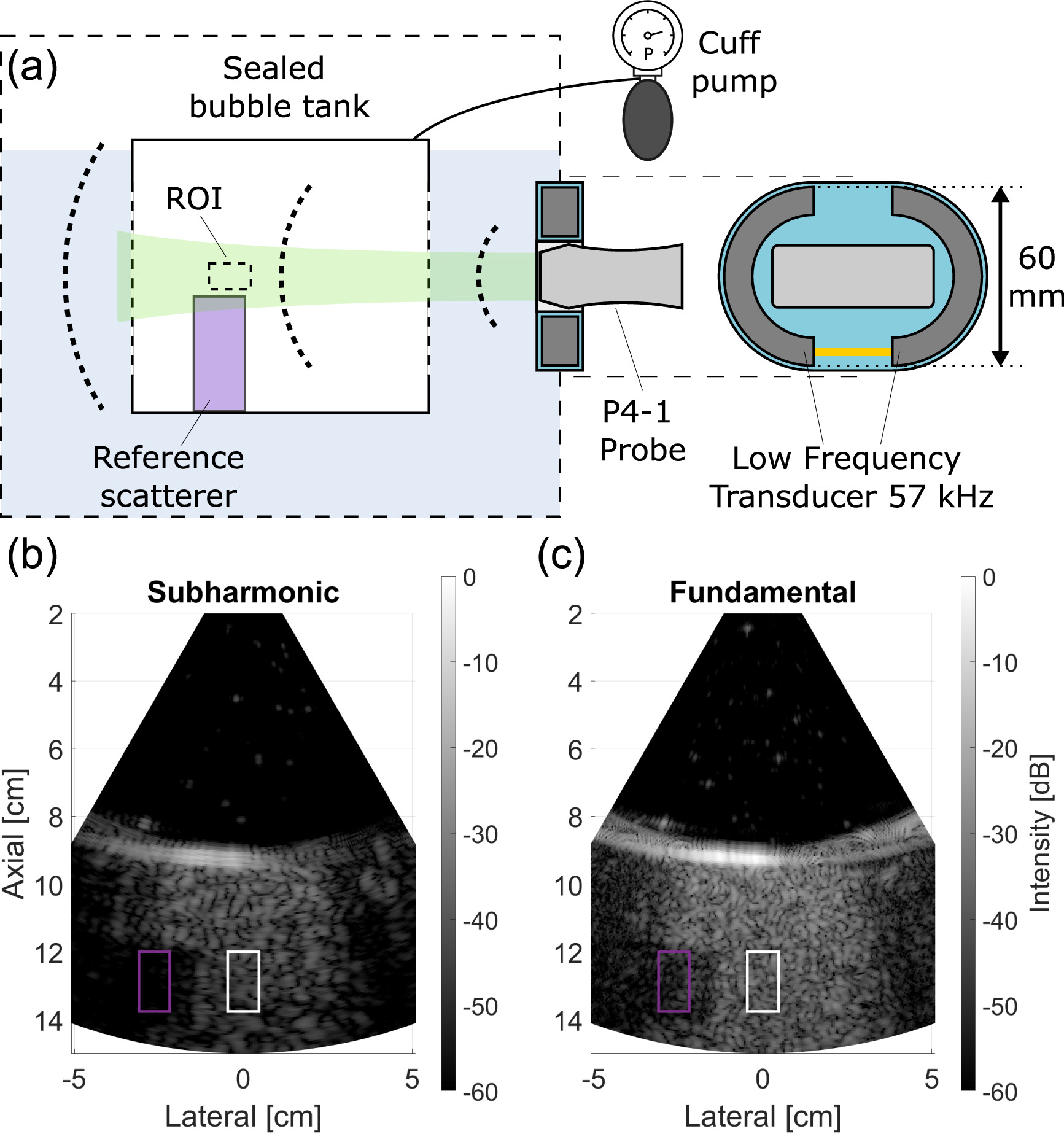
The eight-cycle, 4 MHz imaging pulse was transmitted with a PRF of 100 Hz, with a fixed 38 μs delay from the start of the DAP using an external trigger signal to account for the slower amplitude response of the low-frequency (LF) transducer. Sixteen modulated frames were followed by 16 unmodulated frames, and an additional delay of one tenth of the low-frequency wavelength (1.75 μs) was programmed after each group of 32 frames. After 320 frames the additional delay was reset to zero, and this entire sequence was repeated four times, which resulted in capturing frames with instantaneous ambient pressures that corresponded with the diagram in Figure 4 . The total sequence recorded at each SAP setting encompassed 1280 frames (4 × 320) recorded over 12.8 s. Of these 1280 frames, 640 were DAP modulated and 640 were unmodulated control frames.
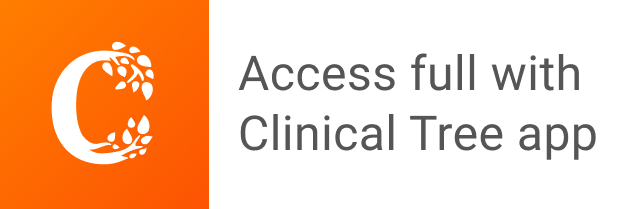