Abstract
Objective
Low-intensity pulsed ultrasound (LIPUS) is a promising modality for neuromodulation. Microglia are the resident immune cells in the brain and their mobility is critical for maintaining brain homeostasis and alleviating neuroimmune pathologies. However, it is unclear whether and how LIPUS modulates microglial migration in physiological conditions.
Methods
Here we examined the in vitro effects of LIPUS on the mobility of BV2 microglia by live cell imaging. Single-cell tracing of BV2 microglia migration was analyzed using ImageJ and Chemotaxis and Migration Tool software. Pharmacological manipulation was performed to determine the key molecular players involved in regulating ultrasound-dependent microglia migration.
Results
We found that the distance of microglial migration was enhanced by LIPUS with increasing acoustic pressure. Removing the extracellular Ca 2+ influx or depletion of intracellular Ca 2+ stores suppressed ultrasound-enhanced BV2 migration. Furthermore, we found that blocking the reorganization of actin, or suppressing purinergic signaling by application of apyrase or hemi-channel inhibitors, both diminished ultrasound-induced BV2 migration. LIPUS stimulation also enhanced microglial migration in a lipopolysaccharide (LPS)-induced inflammatory environment.
Conclusion
LIPUS promoted microglia migration in both physiological and inflammatory environments. Calcium, cytoskeleton, and purinergic signaling were involved in regulating ultrasound-dependent microglial mobility. Our study reveals the biomechanical impact of ultrasound on microglial migration and highlights the potential of using ultrasound-based tools for modulation of microglial function.
Introduction
Cell migration is essential to various physiological and disease-related processes, and development. In particular, mobility is a crucial characteristic of immune cells, enabling them to identify invading infections, manage tissue damage and destroy primary malignancies, even in the absence of external treatment [ ]. Impaired migration of immune cells has been linked to autoimmune disorders, chronic inflammation and tumor evasion [ ]. Microglia are resident immune cells in the central nervous system that monitor the brain microenvironment through highly dynamic cellular protrusions [ ]. Under physiological conditions, microglia actively survey the brain and modulate neuronal activity in a contact-dependent manner [ ]. In response to pathological damage, microglia rapidly move toward sites of injury via purinergic signaling [ , ]. Impaired mobility and motility of microglia can result in seizures, accelerated progression of Alzheimer’s disease and over-excitation of brain networks in ischemic stroke [ , ]. Therefore, maintaining or improving the migratory motility of microglia is critical for preserving the homeostasis of the brain’s internal environment and alleviating neuroimmune diseases.
Ultrasound has recently emerged as a modulator of brain activity [ ], the tumor microenvironment [ ] and bone regeneration [ ]. The unique thermal, mechanical or cavitation effects of focused ultrasound (FUS) can be applied to tumor ablation, blood-brain barrier opening and non-invasive precise control of neuronal activity [ , ]. Pulsed ultrasound waves can produce mechanical pressure to activate mechanosensitive ion channels, receptors and integrins, triggering diverse biological processes such as neuronal firing, cell migration and immune responses [ ]. Recent studies have found that transcranial FUS at 40 Hz is able to activate microglia and alter their morphology [ ]. Low-intensity pulsed ultrasound (LIPUS) promotes the polarization of microglia toward an anti-inflammatory M2 phenotype, enhances the brain-derived neurotrophic factor pathway and reduces the expression of pro-inflammatory cytokines in microglia [ ]. LIPUS could also facilitate microglial migration following injury in vivo [ ]. However, it is not yet clear whether ultrasound can improve microglial mobility under both physiological and pathological conditions, and the molecular and cellular mechanisms by which LIPUS modulates microglial migration remain elusive.
Accumulated evidence implicates the roles of cell surface receptors, calcium (Ca 2+ ) signaling, the actin cytoskeleton and actomyosin in cell migration [ , ]. Microglia express multiple mechanosensitive ion channels such as Piezo1 and thermosensitive transient receptor potential (TRP) channels [ , ]. Both in vitro and in vivo studies have indicated that Piezo1 channels mediate microglial migration, immune response, amyloid beta stiffness mechanosensation and clearance [ ]. TRPV4 has been shown to regulate temperature-dependent microglia movement, as well as the morphology and migratory properties of microglia [ , ]. Although these channels can be activated under specific ultrasound parameters to promote Ca 2+ influx and achieve neuromodulation [ ], it is unclear whether ultrasound regulates microglia migration through these ion channels and Ca 2+ -related signaling pathways.
In this study, we identified that LIPUS improves microglial migration in vitro . We also revealed that extracellular Ca 2+ , mechanosensitive ion channels and endoplasmic reticulum (ER) Ca 2+ release play important roles in LIPUS-induced microglia migration. Additionally, we showed that actin cytoskeleton remodeling, Ca 2+ and purinergic signaling are involved in ultrasound-promoted microglial migration. Furthermore, ultrasound is capable of significantly improving the mobility of BV2 microglia in the inflammatory condition of lipopolysaccharides (LPS). Thus, we found that LIPUS dynamically modulates microglial migration in vitro, which could facilitate the development of ultrasound-based methods for improving microglial function in the future.
Materials and methods
LIPUS system and stimulation parameters
Two connected function generators were used in our pulsed FUS system. The first function generator (Rigol DG972 70 MHz Function/Arbitrary Waveform Generator, Beijing, China) generated a pulse wave, which triggered the second function generator. It controlled pulse repetition frequency (PRF; 10 Hz), the duty cycle (DC) of each pulse (DC = 10%) and sonication duration (= 20 s). The second function generator was used to control the ultrasound fundamental frequency (= 2.25 MHz) and a peak-peak voltage amplitude of 200–400 mV pp . The pulse signal from the second function generator was amplified by a linear power amplifier (2100 L, Electronics and Innovations, Inc., Rochester, NY, USA) and transmitted to a 2.25 MHz FUS transducer with an estimated focal size of 2 mm (Olympus V325-SU-F0.5IN-PTF, Tokyo, Japan). An oscilloscope (Tektronix TBS2000B digital oscilloscope, Oregon, USA) was used to verify the output of the function generator in real time during sonication. The FUS transducer, combined with a coupling cone filled with degassed water, was immersed in the extracellular solution at a 45-degree angle using a micromanipulation system (ROE-200, MPC-200, Sutter Instrument Company, CA, USA). The FUS system and experimental setup are shown in Figure 1 A, and the ultrasound waveforms used in these studies are shown in Figure 1 B.
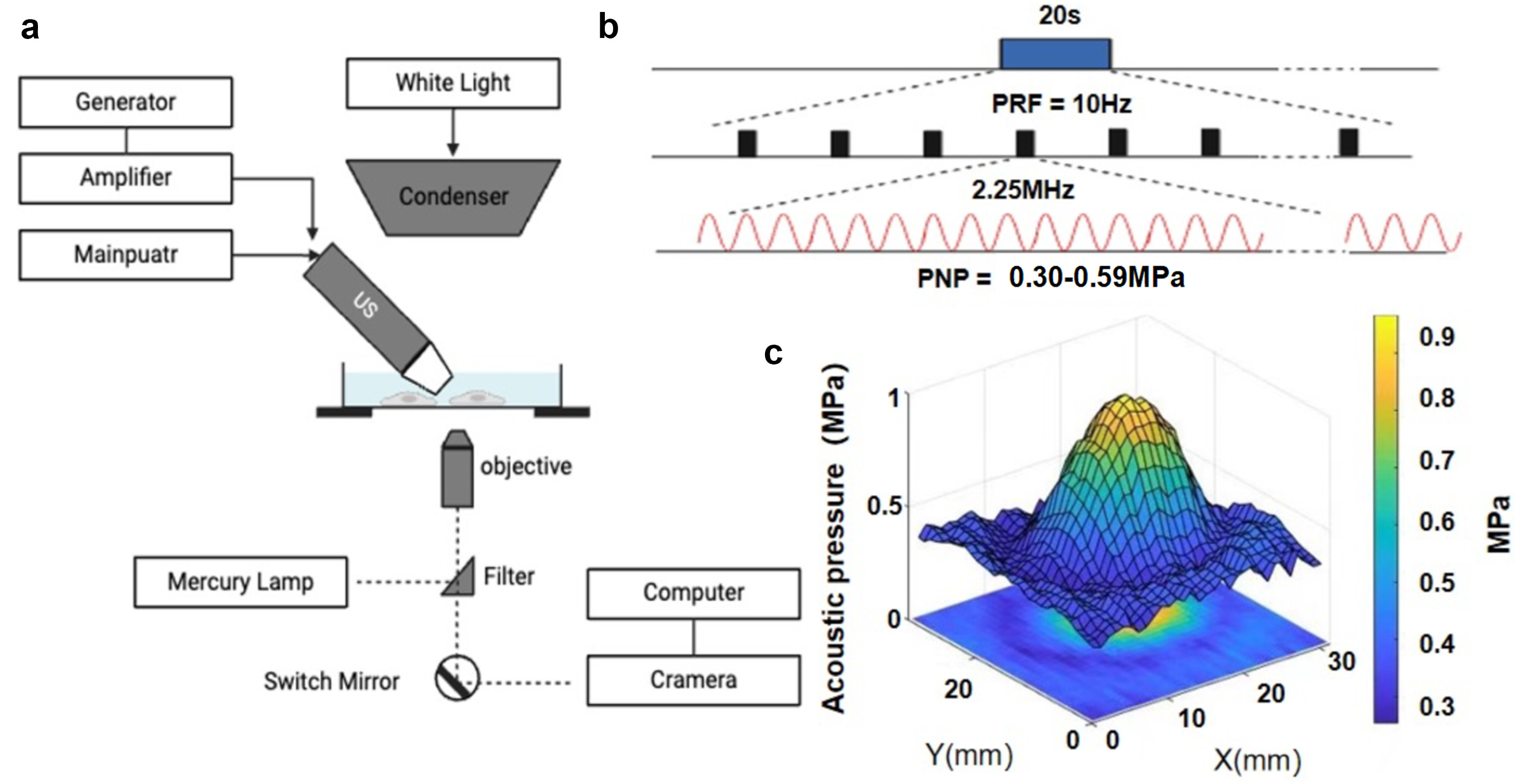
Cell culture
The BV2 cell line used in this study was derived from Procell (CL-0493). BV2 cells were cultured in high-glucose Dulbecco’s modified Eagle’s medium (DMEM) supplemented with 10% fetal bovine serum (FBS) and 1% penicillin/streptomycin solution. Cells were incubated in a humidified atmosphere at 37°C and 5% CO 2 . Once confluence was reached, cells were re-seeded into new flasks. Prior to the experiment, they were re-plated to PDL-precoated sterilized coverslips at low densities for further study. To avoid intercellular Ca 2+ signaling, the cells were seeded at a low concentration of 1 × 10 5 cells/mL.
Reagents
DMEM (C11995500BT), penicillin/streptomycin solution (15140-122) and trypsin (12605-028) were purchased from Gibco. FBS (F8318), Ruthelium Red (RR; 00541), GdCl 3 (439770), thapsigargin (Thaps; T9033), 2-APB (D9754), apyrase (A6535), carbenoxolone (CBX, C4790), GAP19 (SML1426), ML-7 (I2764), LPS (L5293) and poly- d -lysine (PDL, P6407) were from Sigma. YODA1 (HY-18723), GsMTX4 (HY-P1410), GSK101 (HY-19608) and GSK219 (HY-100720) were purchased from MedChemExpress. DPBS (D1040) and DMSO (D8371) were purchased from Solarbio. Iso-PPADS (0683) was purchased from Tocris Bioscience. Cytochalasin D (PHZ1063) was purchased from Invitrogen.
LIPUS treatment of BV2 cells and mechanistic study
The BV2 cells on the coverslip were placed inside a glass-bottomed reservoir filled with 1× DPBS. Cell migration studies were performed using an inverted microscope (Olympus IX73) with an sCMOS camera (PCO edge 4.2). To study the mechanism of ultrasound-induced BV2 cell migration, cells were exposed to 10 μM RR, 100 μM GdCl 3 , 10 μM Thaps, 10 μM GsMTX4, 30 μM YODA1, 1 μM GSK101, 30 μM GSK219, 20 U apyrase, 100 μM 2-APB, and 100 μM CBX and 20 μM GAP19 in 1× DPBS, respectively. The above reagents were initially dissolved in DMSO or 1× DPBS and then diluted to the target concentrations in 1× DPBS, with 1× DPBS containing the same volume of DMSO serving as a control. Cells were stimulated with pulsed FUS for 20 s. Bright-field images were acquired every 1 min to track cell migration by Micro-Manager with a 40× objective (Olympus, LUCPlanFL N, NA 0.60) for up to 30 min; a previously reported migration velocity of BV2 cells cultured in microfluidic channels with a PLL-coated glass substrate at high density was approximately 11 µm/h [ ]. In order to track individual BV2 cell mobility by live imaging with a measurable migration distance, we chose 30 min instead of a shorter period of time. Additionally, to maintain minimum LIPUS-independent disturbance of the cells in media, we did not remove the transducer from the glass-bottomed reservoir. Therefore, the cells were not maintained in a closed culture environment. To keep the cells viable and suitable for biological characterization, we set the observation time as 30 min instead of hours.
Ca 2+ imaging
Cells were seeded on PDL-coated cover glass and incubated in DMEM at 37°C for 3 h to facilitate cell adhesion and spreading. The cells were then incubated at 37°C with 5% CO 2 in DMEM containing 0.5 µM of Fluo-8 AM (AbMole BioScience, M15051) for 45 min. Excessive Fluo-8 AM was washed with DMEM and then incubated in DMEM for 15 min. Cells were monitored in real time with Ca 2+ imaging for 30 min with a 3 s time interval.
Chemotaxis assay
LPS was continuously injected into the cell sample at a rate of 0.2 μL/min for 30 min through a micropipette with a microinjection system (KDS LEGATO 130). The micropipette tip was placed at the center of the imaging field to generate a chemoattractant gradient with the slow release of LPS. The migration of cells was monitored by bright-field imaging every 1 min for 30 min.
Data analysis
The migration paths of individual cells were extracted from bright-field images, and the total distance of cell migration was measured using Chemotaxis and Migration Tool software. The averaged migration velocity of each cell was calculated from the total distance of migration and the corresponding time. Ca 2+ imaging data were analyzed using a customized MATLAB code. Fluorescent images were pre-processed by filtering and adjusting the grayscale histogram. Subsequently, the Canny algorithm was employed to accurately detect the cell edges, separating the cells from the background. A Kalman filter was used to trace individual cell motion. The fluorescent intensity, F, was measured for each cell over time. The baseline fluorescent intensity, F 0 , was identified as the average fluorescent intensity of the first six frames before ultrasound stimulation. The relative change in fluorescence intensity was then calculated as (F – F 0 )/F 0 . The MATLAB built-in function findpeaks was used to determine the maximum Ca 2+ response amplitude (F max – F 0 )/F 0 , Ca 2+ response frequency and the area under the curve (AUC). Statistical analyses were performed using GraphPad Prism. Data were presented as mean ± SD, where applicable. Significant differences were determined by one-way or two-way analysis of variance (ANOVA) with multiple-comparison tests; *p < 0.05, **p < 0.001 or ***p < 0.0001.
Results
Experimental system and acoustic field characterization
To monitor the effects of LIPUS stimulation on microglial migration, we developed a customized in vitro ultrasound stimulation system incorporated with an inverted epifluorescence microscope ( Fig. 1 a). An ultrasound wave was incident at a 45-degree angle to stimulate the cells. Each ultrasound stimulus was delivered at a center frequency of 2.25 MHz, a PRF of 10 Hz, 10% DC, a peak negative pressure of 0.30–0.59 MPa and a total treatment time of 20 s ( Fig. 1 b). The corresponding spatial peak pulse average intensities ranged from 0.31 to 1.16 W/cm 2 , which fell within the low-intensity range. The acoustic beam profile and the pressure output of the transducer were measured using a needle hydrophone (Onda, HNR-0500, CA, USA) in a tank with degassed water. The measured acoustic pressure is demonstrated in Figure 1 c. To mitigate the heating effect of LIPUS, a low DC of 10% was used in our experiments. We also measured the temperature change in the absence and presence of LIPUS treatment under different acoustic pressures. Compared with a 0.2°C variation in the control group, minimum temperature changes (0.4–0.6°C) were found in the LIPUS group ( Supplementary Figure 1 ).
LIPUS promotes microglia migration
To examine if LIPUS was able to affect microglial migration, we traced individual BV2 cells throughout ultrasound stimulation by bright-field imaging. BV2 microglia are usually round or spindle-shaped with short tentacles or pseudopods protruding. The migratory movement of cells is accompanied by gradual changes in cell morphology. After LIPUS stimulation, a significant increase in the accumulated distance of BV2 cell migration was observed, accompanied by noticeable changes in cell morphology, including the presence of protruding or retracted cell pseudopods ( Fig. 2 a). Next, we tested whether this increase in microglial migration was affected by ultrasound parameters, including different acoustic pressures, DCs and PRFs. Overall, the accumulated distance of cell migration increased with higher acoustic pressures ( Fig. 2 b), larger DCs ( Fig. 2 c) and elevated PRFs ( Fig. 2 d) over the 30 min recording time. Statistical analysis revealed that the total migration distances of the cell population were significantly higher under LIPUS stimulation at 0.30, 0.43 and 0.59 MPa acoustic pressure with 10% DC and 10 Hz PRF compared with the control group without ultrasound stimulation ( Fig. 2 e). Similar results were observed under LIPUS stimulation at 20% and 30% DCs with 0.43 MPa acoustic pressure and 10 Hz PRF ( Fig. 2 f), or by changing the PRF from 10 Hz to 100 Hz and 1000 Hz while maintaining 0.43 MPa acoustic pressure and 10% DC ( Fig. 2 f). We thus confirmed that LIPUS stimulation promotes microglia migration. We chose LIPUS of 0.43 MPa with 10% DC and 10 Hz PRF for further mechanistic studies, as LIPUS at this setting can significantly enhance the accumulated migration of BV2 while the low DC and PRF minimize thermal effects ( Supplementary Fig. 1 ). No propidium iodide (PI) uptake was observed in BV2 cells at this ultrasound condition ( Supplementary Fig. 2 ).
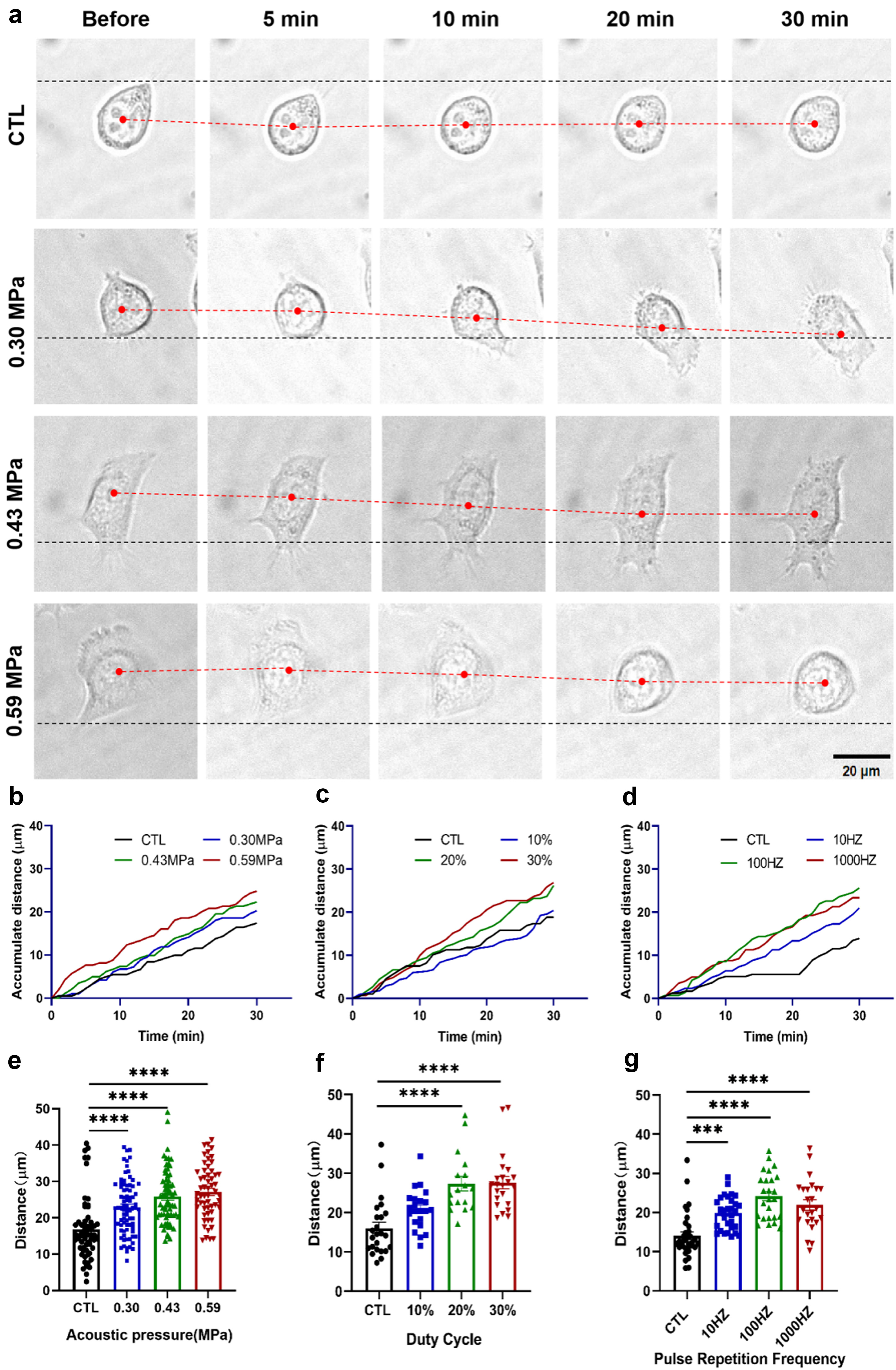
Extracellular Ca 2+ , mechanosensitive ion channels and ER Ca 2+ release are involved in enhanced LIPUS microglial migration
Ca 2+ signaling has long been known to play important roles in regulating cell migration [ ]. It has been reported that microglia podosome formation and migration require Ca 2+ influx and Ca 2+ channels [ ], and the increased Ca 2+ transient is associated with process extension and microglial motility [ , ]. To examine if extracellular Ca 2+ is required for ultrasound-promoted migration in microglia, we substituted the BV2 cell extracellular medium with 1× DPBS devoid of Ca 2+ and Mg 2+ . The total distance of migration in the LIPUS-treated group decreased in the Ca 2+ -free external solution ( Fig. 3 a–c). Interestingly, the exemplary accumulated migration trace of ultrasound-stimulated BV2 cells in the absence of extracellular Ca 2+ plateaued at 20 min, while the accumulated migration distance of ultrasound-stimulated BV2 cells in the presence of extracellular Ca 2+ continued to increase. This result suggests that ultrasound could initiate cell migration for short periods of time (less than 20 min), even in the absence extracellular Ca 2+ , but requires extracellular Ca 2+ for continuous movement. In the control groups without ultrasound stimulation, the presence or absence of Ca 2+ ions in the external solution did not have a significant impact on the total migration distance of the cells ( Fig. 3 c). These results imply that extracellular Ca 2+ plays an important role in regulating ultrasound-facilitated microglial migration.
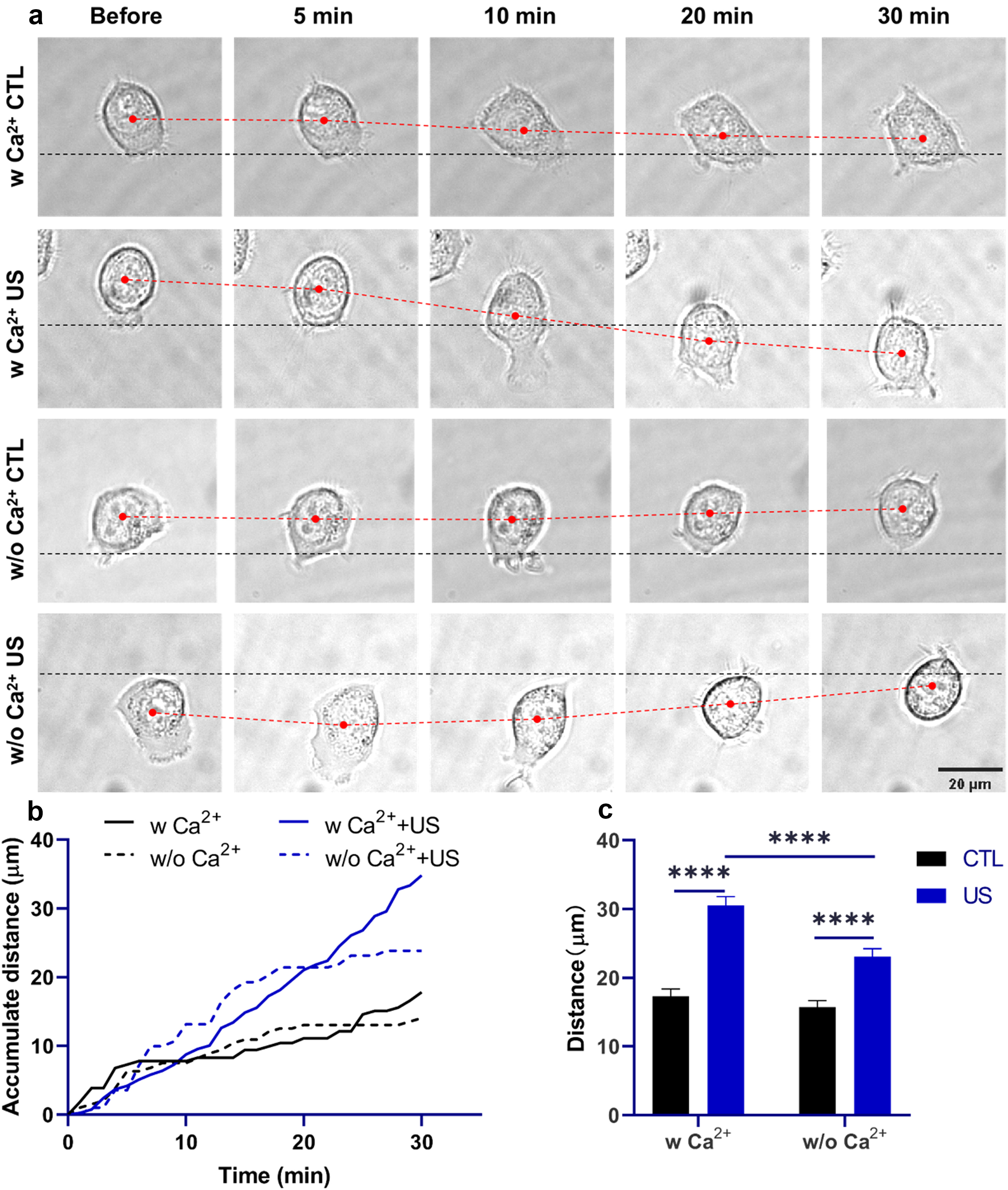
Extracellular Ca 2+ may enter cells through mechanically activated ion channels with ultrasound stimulation [ , , ]. To explore the roles of cation channels in regulating ultrasound-facilitated microglial migration, we first applied RR, a universal blocker for a wide array of cation channels, and then GdCl 3 , a cation-selective stretch-activated channel antagonist, to BV2 cell cultures. The promoted migration of BV2 cells by ultrasound was notably suppressed in the presence of RR or GdCl 3 ( Fig. 4 a–d). Additionally, RR but not GdCl 3 decreased the normal migratory activity of BV2 cells in the absence of ultrasound stimulation ( Fig. 4 b, 4 d), suggesting that cation-selective stretch-activated channels are more likely required for ultrasound-dependent increase of microglial migration. We further tested the roles of two mechanosensitive ion channels that were reported to be expressed in microglia [ , , ]—Piezo 1 and TRPV4—in the ultrasound-induced migration of BV2 cells. Treating BV2 cells with Piezo1 inhibitor GsMTX4 suppressed ultrasound-induced cell migration, whereas treatment with Piezo1 agonist Yoda1 slightly but not significantly increased baseline microglial migration ( Fig. 4 e–g). However, TRPV4 inhibitor GSK219 did not suppress microglial migration when subjected to ultrasound stimulation, while TRPV4 agonist GSK101 significantly promoted the baseline migration of BV2 cells in the absence of LIPUS, comparable to the effects of ultrasound stimulation ( Fig. 4 h, 4 i). These results indicate that the mechanosensitive ion channel Piezo1, instead of TRPV4, plays an important role in facilitating the migratory behavior of microglia in response to ultrasound stimulation.
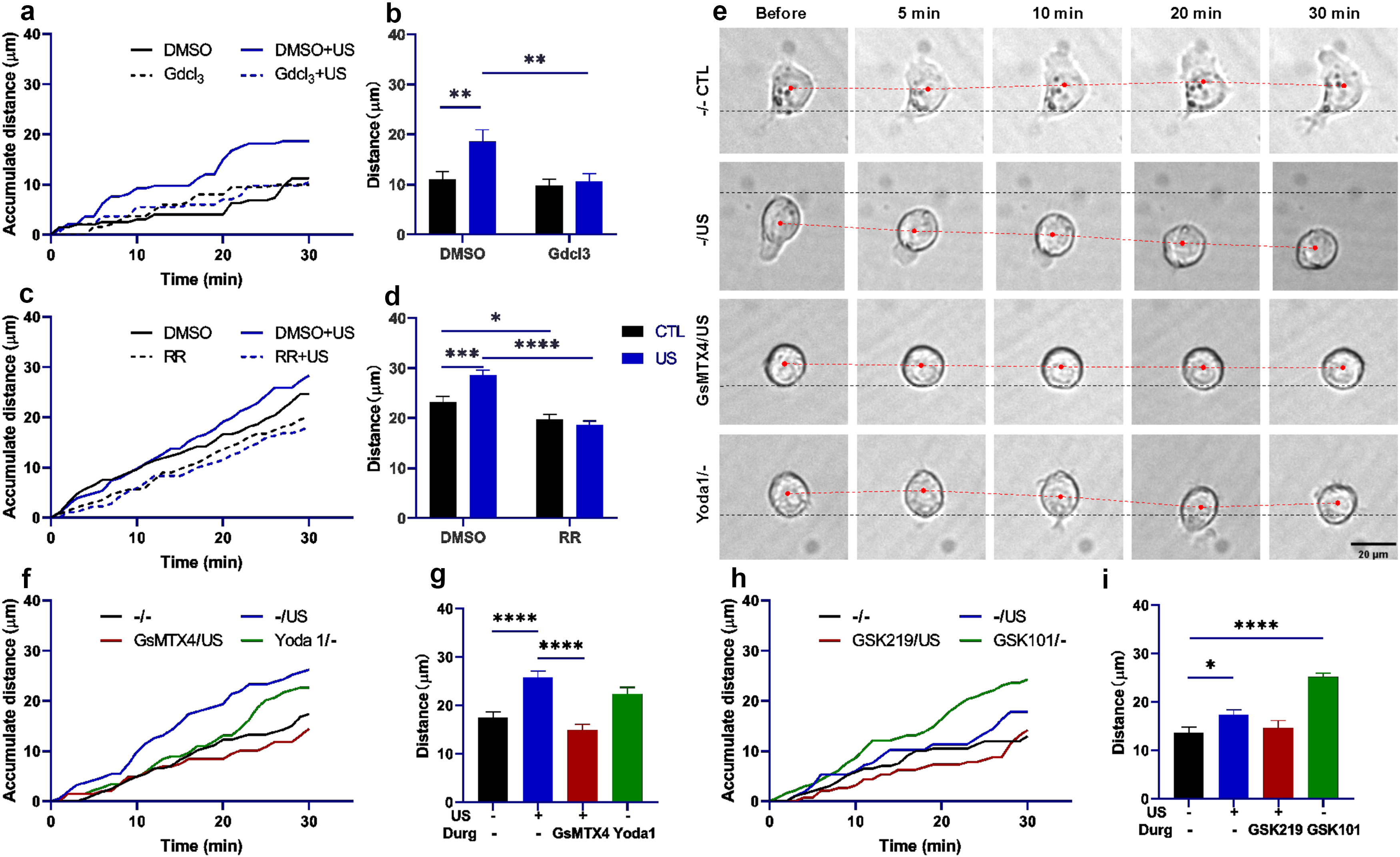
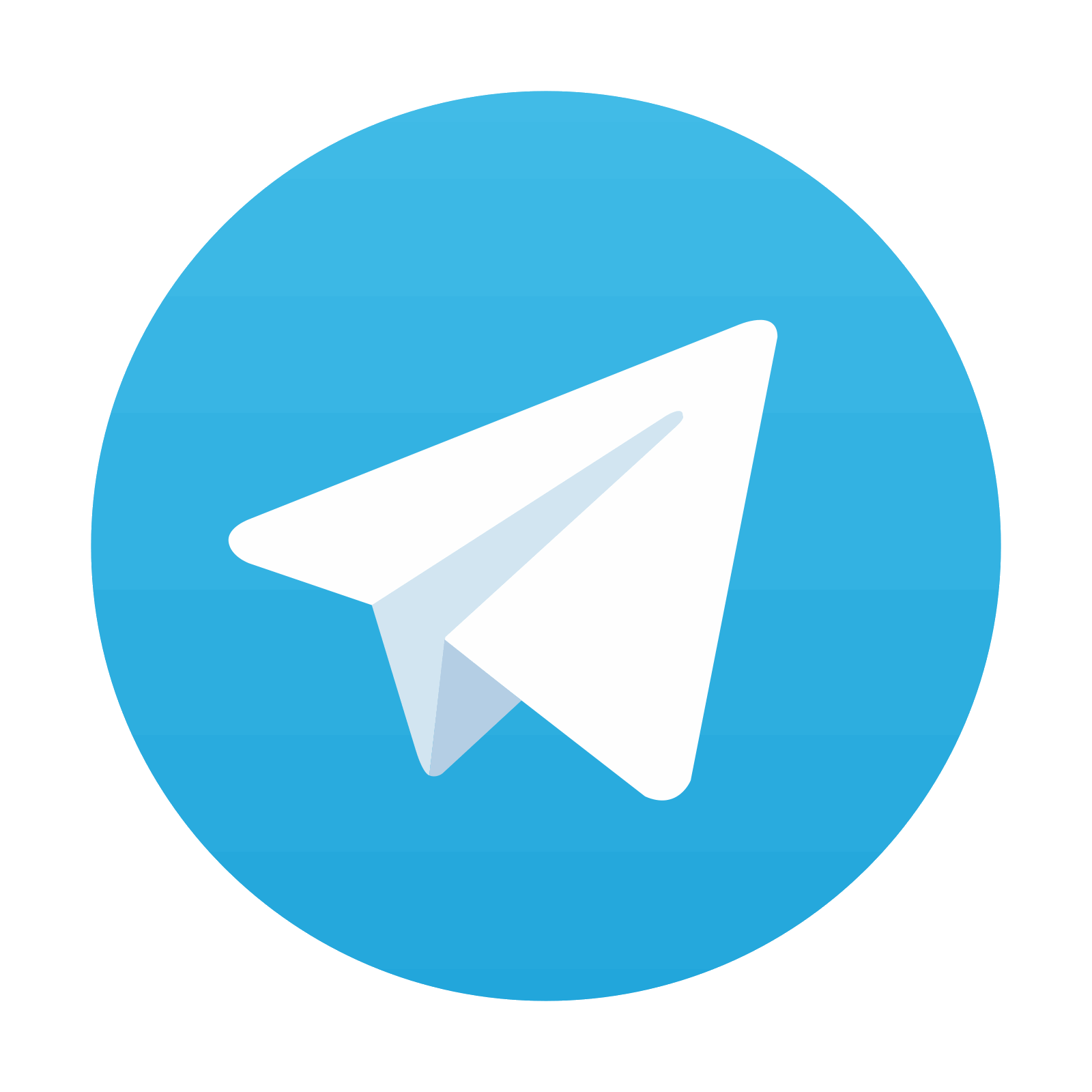
Stay updated, free articles. Join our Telegram channel
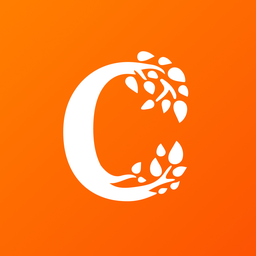
Full access? Get Clinical Tree
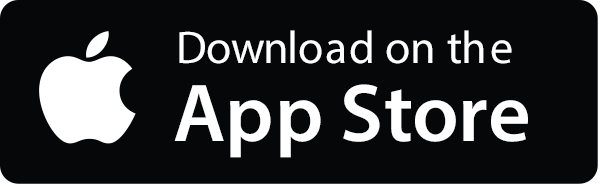
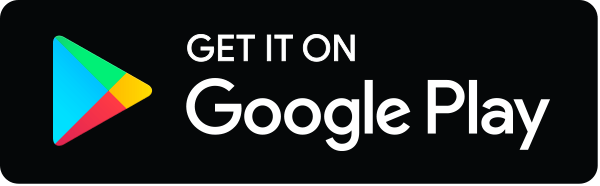
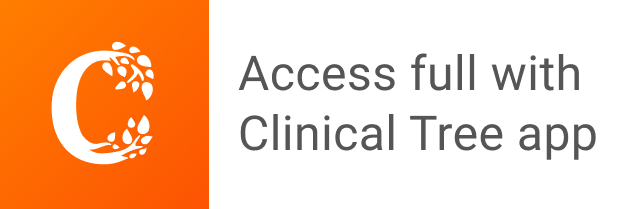