Abstract
Objective
Nasal-to-brain (NtoB) delivery is a noninvasive approach that uses the nasal cavity as a pathway to transport therapeutic agents directly to the brain. This approach bypasses systemic circulation and avoids the blood-brain barrier (BBB). Transcranial ultrasound, coupled with microbubbles (MB), is a technique used to oscillate and generate acoustic cavitation to open the capillary tight junctions of BBB temporarily. Its efficacy in facilitating NtoB delivery has been demonstrated in vivo. However, while opening the BBB, sonication with MB poses the risk of cerebral microhemorrhage or brain tissue damage due to sonication-induced physical injury. This study aimed to assess the effectiveness of low-intensity ultrasound treatment to facilitate NtoB delivery in a mouse model without using MB.
Methods
In this study, 10-kDa dextran was administered intranasally (IN), and transcranial planar US was applied to the entire mouse brain without MB assistance. Ex-vivo whole brain imaging via fluorescence macroscopy, brain slice analysis with fluorescence microscope, and quantification of dextran concentration in distinct brain regions were conducted to compare the IN-only, IN combined with US (IN+US), and sham groups. For the trigeminal nerves (TN), fluorescence macroscopy, microscopy, and TN concentration quantification were performed to compare the three groups.
Results
Whole brain imaging revealed that US facilitated the IN delivery of dextran to the olfactory bulb (OB) in the IN+US group compared with that in the IN-only and sham groups; however, this difference was not observed after a 24 h follow-up. Conversely, brain slice images showed that the tracer was delivered to the OB, cerebral cortex, striatum and brainstem in the IN+US group, but this finding was not observed in the IN-only group at the 4 h mark. The quantification of fluorescence intensity at two follow-up time points revealed no significant difference between the IN and IN+US groups in these specific regions. Dextran concentration analysis for distinct brain areas and TN showed that ultrasound significantly increased the tracer concentration delivered to the OB and TN in the IN+US group at the 4 h mark compared with that in the IN-only and sham groups; however, this effect was not sustained at 24 h. Confocal microscopy indicated that the dextran tracer accumulated in the perivascular space along the microvascular structures.
Conclusion
We demonstrated the efficacy of low-intensity ultrasound without using MB, in enhancing nose-to-OB and nose-to-TN drug delivery, and proposed the potential for future clinical application. Thus, we showed that this approach was safe, without evidence of microhemorrhage or brain tissue damage.
Introduction
The incidence of central nervous system (CNS)-related diseases, such as brain tumors, Alzheimer’s, and Parkinson’s, is gradually increasing because of an aging population, posing considerable challenges. Current treatments include radiation therapy and surgery for brain tumors; however, they pose risks of damaging healthy tissues. Deep brain stimulation for Parkinson’s disease is an invasive procedure and is limited to targeting specific brain regions. Medications for the treatment of CNS diseases are hindered by the blood-brain barrier (BBB), which protects the brain but restricts drug delivery. The BBB is composed of junctional complexes, including tight, adherens, and gap junctions between endothelial cells, which strictly regulate permeability [ ]. To overcome the challenge of delivering drugs to the brain, researchers introduced nasal administration as a non-invasive method to bypass the BBB [ ]. The nasal cavity is lined with a mucosal membrane rich in blood vessels and nerves. It houses specialized olfactory neurons in the upper region, which directly connect to the olfactory bulb in the brain via the cribriform plate [ ]. The trigeminal nerve (TN), which is responsible for sensory and autonomic functions, is distributed throughout the nasal cavity, influencing neurological and vascular responses [ ]. Because of the dense network of blood vessels, nerves, and its proximity to the brain, the nasal cavity is a crucial target for therapeutic approaches in medicine. Although the nasal mucosa is highly vascularized and thus allows medications to enter the bloodstream, these drugs face difficulty reaching the brain because of the BBB; they are quickly eliminated through first-pass metabolism [ ]. Intranasal (IN) drug delivery offers the advantages of convenient administration and rapid onset of effect. It primarily relies on transportation through the olfactory nerve and TN via intracellular and extracellular pathways [ , ]. The intracellular pathway involves endocytosis and transport within olfactory sensory cells, while the extracellular pathway is the paracellular route of drug from the nasal epithelium to reach the perineural space of the olfactory bulb and access the subarachnoid space through the cerebral spinal fluid (CSF) [ ]. Drugs entering the CSF are propelled by arterial-generated pressure waves within the perivascular space (PVS), known as the glymphatic system, facilitating rapid distribution throughout brain tissue [ ]. Several medications, including cocaine, insulin and interferon β, for IN have been studied to attempt to bypass the BBB [ , ]. In recent years, peptides, proteins, nucleic acids, and even stem cells have been administered via the nasal route; in addition to small molecules and macromolecules, nanoparticles for IN drug delivery have been widely explored [ ].
To enhance the delivery of drugs into the brain, researchers explored various strategies such as mechanical stimulation. Evidence revealed the potential of focused ultrasound (FUS) to open the BBB and facilitate IN drug delivery while minimizing systemic exposure. One study showed the successful localized delivery of gold nanoclusters to the pons by using FUS for IN delivery [ ]. In a brain tumor model, the accumulation of anti-programmed cell death-ligand 1 antibody at the FUS-targeted brainstem significantly increased [ ]. The nasal delivery of a brain-derived neurotrophic factor via FUS demonstrated that motor control in a Parkinson’s disease mouse model improved [ ]. However, using FUS to open the BBB is accompanied by potential bleeding and tissue damage; it is dependent on microbubbles (MB) to generate cavitation for BBB opening [ ]. Transcranial FUS without using MB showed the effectiveness for the CSF influx penetrating the brain parenchyma to preserve intact BBB [ ]. In an ischemic stroke animal model, FUS without MB protects brain tissue, as indicated by behavioral and histological evidence [ ]. Our previous research demonstrated that low-intensity plantar ultrasound, without MB, is safe and capable of increasing the flow of the CSF into the PVS of the brain [ ]. This finding has potential implications for applying low-intensity ultrasound in IN drug delivery systems.
Therefore, this study aimed to investigate the efficacy of low-intensity plantar ultrasound (US) without MB in delivering drugs to the brain via the nasal route. This study would be the first to utilize low-intensity ultrasound without contrast agents in NtoB research. We hypothesized that low-intensity plantar US, without MB, might enhance drug delivery to the brain and the TN in our animal model.
Methods
Animal model
A total of 95 C57BL/6JNarl mice, aged 7–9 weeks and weighing 20–30 grams, were used in our study ( Table 1 ). Their health was thoroughly assessed, and they were allowed to adapt to the experimental environment before administration. This research was performed in accordance with the Institutional Animal Care and Use Committee guidelines of the University (approval number 20230261). The experiments ( Table 1 ) were conducted to compare the IN-only group, the IN-and-US (IN+US) group, and the sham group.
Experiments | Groups | Analysis |
---|---|---|
Experiment 1 | IN (N= 11) vs. IN+US (N= 11) vs. Sham (N=5) at 4 hours IN (N= 8) vs. IN+US (N= 8) vs. Sham (N=3) at 24 hours | Whole brain imaging ( Fig. 2 ) |
Experiment 2 | IN (N= 3) vs. IN+US (N= 4) at 4 hours IN (N= 3) vs. IN+US (N= 3) at 24 hours | Brain slice Image analysis ( Fig. 3 ) |
Experiment 3 | IN (N= 7) vs. IN+US (N= 7) vs. Sham (N=5) at 4 hours IN (N= 5) vs. IN+US (N= 5) vs. Sham (N=3) at 24 hours | Tracer concentration of brain areas ( Fig. 4 ) |
Experiment 4 | IN+US (N= 1) at 4 hours | Immunofluorescence staining ( Fig. 5 ) |
Experiment 5 | IN (N= 1) vs. IN+US at 4 hours (N= 1) and at 24 hours (N= 1) | H&E staining ( supplement figure 1 ) |
Nasal dextran delivery
In the IN delivery, substances were precisely instilled through the nostrils for targeted administration and absorption. Then, 10-kDa dextran (conjugated with Alexa Fluor™ 555, Invitrogen) was prepared at a concentration of 10 mg/mL. IN delivery was conducted on the mice anesthetized by intraperitoneally injecting Zoletil (50 mg/kg) and Xylazine (2.5 mg/kg). The mice were positioned upright using a support apparatus, and 4 µL of the dextran solution was carefully administered to one nostril at a time, alternating between the left and right nostrils ( Fig. 1 a, left). Dextran was IN administered using a 10μL tip (Rainin™; Fig. 1 a, right). The solution was delivered to each nostril for 2 min, with a total delivery of up to 40 μL of dextran. Care was taken to ensure that dextran did not spill out of the nasal cavity throughout the procedure. After dextran was IN administered, the mice were kept in an upright position for 30 min ( Fig. 1 a, b) and fixed in a stereotaxic frame (stereotaxic Instrument 18-7216, BioMed Easy Technologies Co., Ltd) for ultrasound treatment.
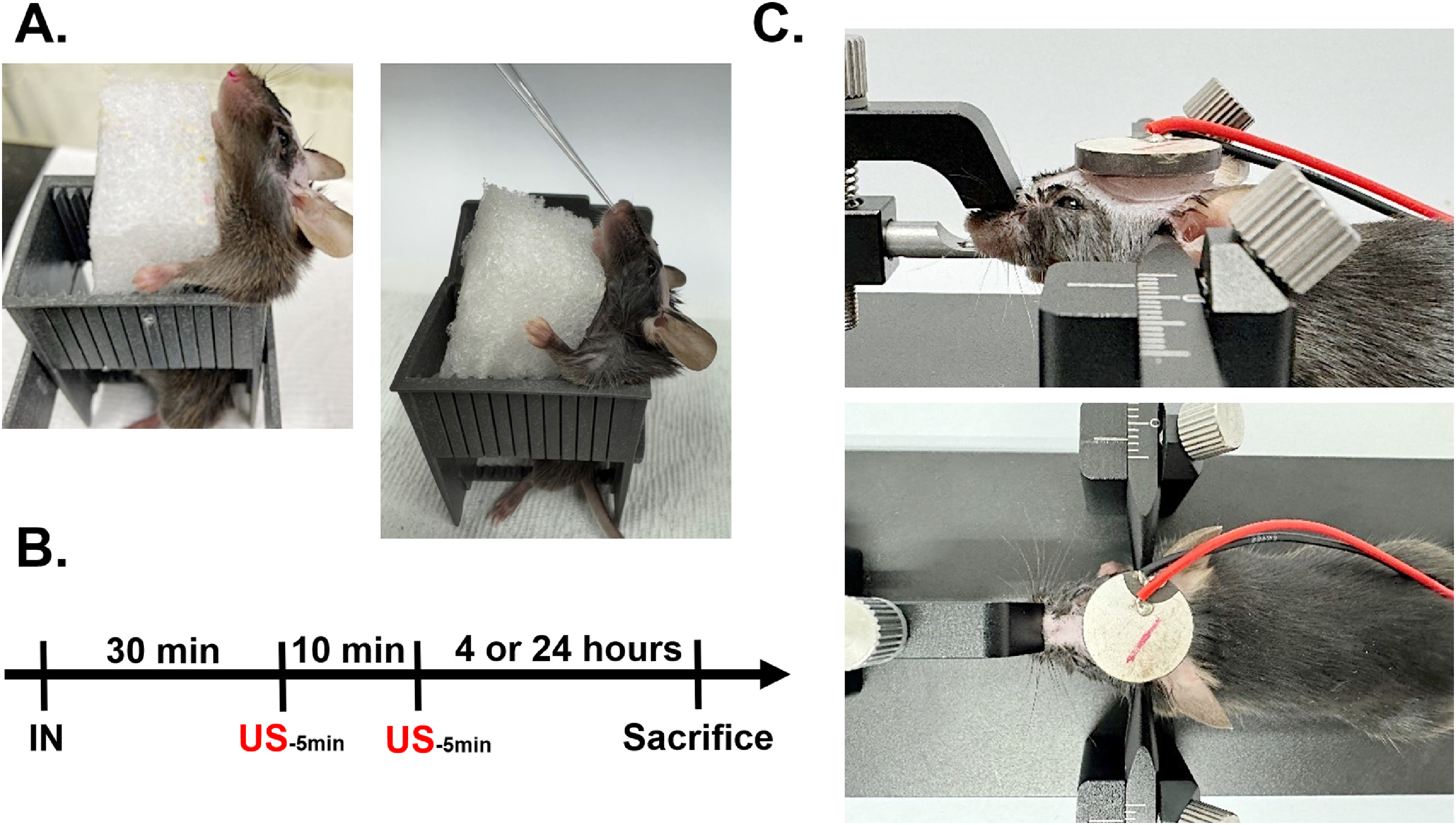
Transcranial ultrasound treatment
The mice were subjected to whole-brain ultrasound treatment twice with 10-min intervals using a self-made 1 MHz planar ultrasound transducer ( Fig. 1 b) [ ]. The head fur was shaved and the scalp was exposed; ultrasound gel was applied between the transducer and the sonicated area at the center of the brain ( Fig. 1 c). The ultrasound transducer was positioned at the midpoint of the line connecting the mouse’s ears and eyes, with the transducer oriented parallel to the exposed skull. This custom-made ultrasound transducer was prepared with a diameter of 15mm based on our previous publications [ , ], and the pressure field of the transducer was measured with a 2.0 mm calibrated needle hydrophone (Precision Acoustics, Dorchester, UK) in the free field within the degassed water ( Supplement Fig. 1A ). The output acoustic pressure (kPa) and intensity of spatial-peak temporal average (Ispta) at the applied input voltage (mVpp) were measured. Before the in-vivo experiment, three ex-vivo conditions were tested using a needle hydrophone to assess the attenuated transcranial acoustic pressure: (1) ultrasound gel alone, (2) the scalp and skull, and (3) the whole mouse head ( supplementary fig. 1B ). The transcranial propagation analysis revealed a 10.4% reduction in acoustic pressure when comparing transmission under condition 2 (scalp and skull) to ultrasound gel alone; additionally, a reduction of 65.9% was observed when comparing condition 3 (whole mouse head) to ultrasound gel alone ( supplement fig. 1C ). Three ex-vivo experiments showed no increase in temperature. Ultrasound was generated using a function generator (Tektronix AFG1022, USA) paired with a power amplifier (E&I 210L, Electronics & Innovation, USA). The parameters were based on previously published settings for the low-energy ultrasound treatment of the whole brain: center frequency = 1 MHz, pulse repetition frequency = 1 kHz, duty factor = 1%, Ispta = 3.68 mW/cm² (equal to pressure level 100 kPa), duration of each stimulation = 5 min [ , ]. Contrast agents were not utilized in our study.
After anesthesia, the mouse’s abdomen and ribcage were carefully opened to expose the heart and liver. A needle was inserted into the left ventricle, and a small incision was made in the right atrium to allow blood to flow out. The circulatory system was initially perfused with physiological saline to flush out blood, as indicated by a pale liver; it was subsequently perfused with a formalin solution (Sigma-Aldrich, HT501128) to fix the tissues. Once perfusion was complete, the brain was harvested for further analysis. The mice were euthanized at 4 and 24 h post-IN administration.
Analysis of the efficiency of nasal drug delivery
Whole brain imaging (Experiment 1)
Before the brain was sectioned, the fluorescence intensity of the mouse brains was imaged from dorsal and ventral perspectives under a fluorescence macroscope (Cat No. MVX10, Tokyo, Olympus) equipped with an ORCA-381 Spark digital CMOS camera (Cat No. C11440-36U, Hamamatsu, Japan). Images of the excised TN were captured under consistent exposure settings throughout the entire imaging session and from all experimental groups ( Fig. 2 a).
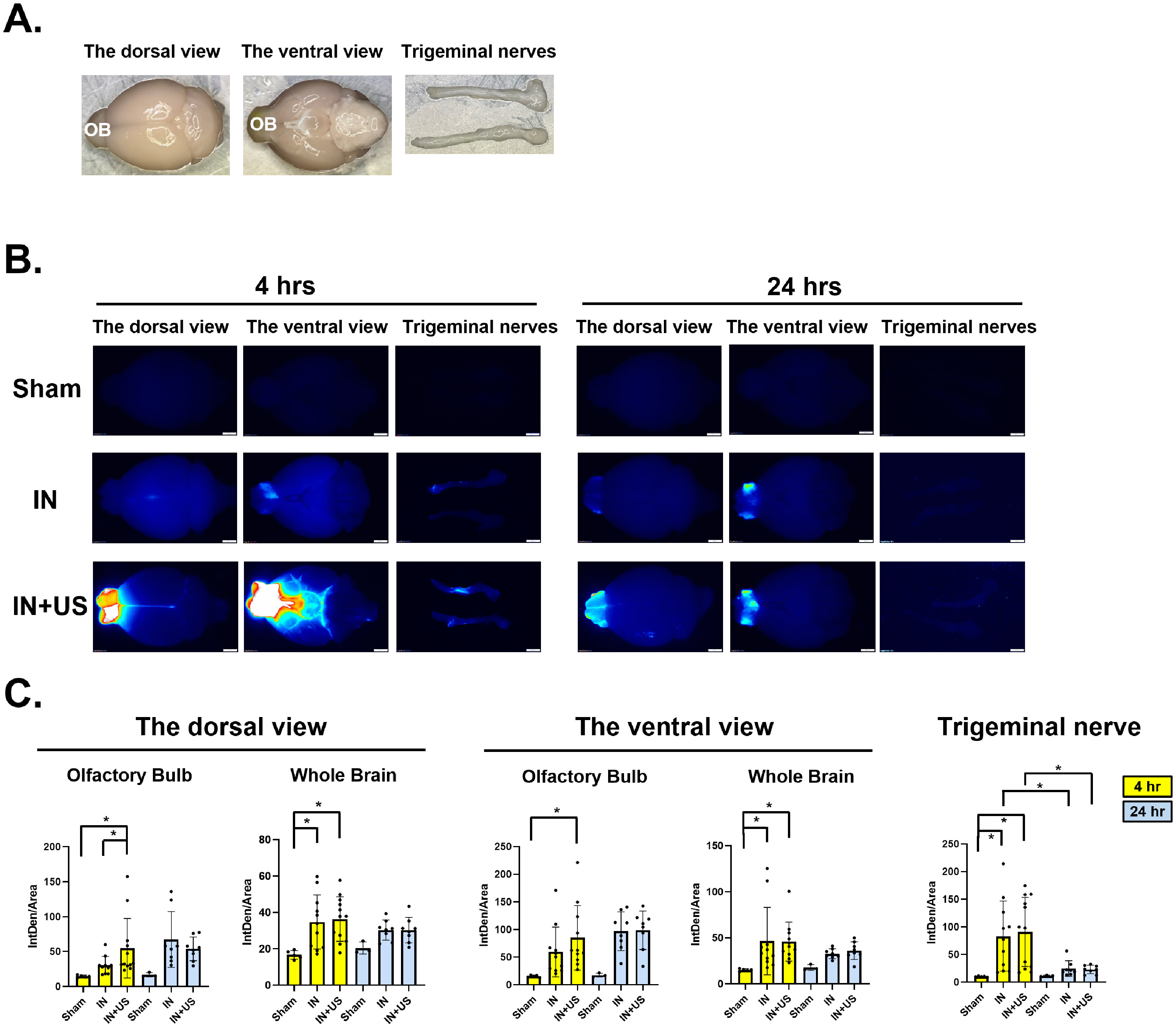
Brain slice imaging analysis (Experiment 2)
Mouse brains were sectioned and analyzed for the distribution of dextran (10-kDa)- Alexa Fluor™ 555 within the brain parenchyma and in neural and vascular network structures. The mouse brain sections were sliced utilizing a vibratome (MicroSlicer™ DTK-1000N, DSK) at a thickness of 500 μm. The brain slices were cleared by incubating in PBS+2% Triton X-100 for 3 days to improve tissue permeability and remove lipids. They were washed with PBS and immersed in RapiClear® clearing reagent for 1 h before they were mounted. Images of the brain slices, including TN treated with the same clearing protocol, were captured via fluorescence microscopy (Olympus BX51). They were analyzed using ImageJ software. The tracer infiltration area or fluorescence intensity was quantitatively analyzed under uniform imaging conditions with consistent threshold settings.
Dextran concentration analysis of distinct brain areas and TN (Experiment 3)
After the mice were euthanized, their brains were removed and dissected into several distinct regions in accordance with established methods ( Fig. 4 a): the olfactory bulb, cerebral cortex, brainstem (including the midbrain, pons, and medulla), cerebellum, and other areas (thalamus and hippocampus) [ ]. Each dissected brain tissue was homogenized with RIPA buffer (Thermo Scientific™). The homogenates were centrifuged at 13,000 × g and 4°C for 30 min. The fluorescence intensity of the supernatant was measured (excitation/emission 535/600 nm in Alexa Fluor™ 555 experiment) with a microplate spectrophotometer (Infinite M200, Tecan, Austria) and normalized to the total protein to obtain relative fluorescence values [ ].
Immunofluorescence staining (Experiment 4)
Brain sagittal slices (500 or 100 μm) were incubated in 2% PBST (2% Triton X-100 in PBS) at 25°C for 3 days, and washed in PBS thrice at 25°C for 15 min per wash. The slices were blocked (blocking buffer: 10% goat serum, 1% Triton X-100, 2.5% DMSO, 0.05% sodium azide in PBS) at 4°C for 3 days. The slices were incubated with a primary antibody (antibody dilution buffer: 1% goat serum, 0.2% Triton X-100, 2.5% DMSO, 0.05% sodium azide in PBS) at 4°C for 3 days. The sections were washed thrice with the buffer (3% NaCl, 0.2% Triton X-100 in PBS) at 25°C for 1 h, and then incubated at 4°C overnight. Afterward, they were incubated with a secondary antibody at 4°C for 3 days. They were washed thrice with a washing buffer at 25°C for 1 h, incubated at 4°C overnight, treated with RapiClear® 1.49 for 1 h, and mounted on slides. For the endothelial cell marker, CD31 (PECAM1) was labeled using a primary CD31 mouse monoclonal antibody (OriGene, AM32708PU-N) at a 1:100 dilution, and with a secondary goat antimouse antibody conjugated with Alexa Fluor™ 633 (Invitrogen, A-21050) at a 1:200 dilution. Imaging was performed using a laser scanning confocal microscope (Zeiss, Jena, Germany), and the images were analyzed with ImageJ software.
Hematoxylin and eosin (H&E) staining (Experiment 5)
H&E staining was performed on brain sections to identify potential vessel-injury-related erythrocyte extravasation or ischemia-associated cell necrosis and to evaluate the safety of the ultrasound treatment. The following regions were selected for analysis: the olfactory bulb, cerebral cortex, and thalamus. Whole brain tissue blocks were embedded in paraffin, sectioned sagittally at a thickness of 4 μm, and subjected to H&E staining with Tissue-Tek Prisma® Plus Automated Slide Stainer (Sakura™, Nagano, Japan) for histological assessment.
Statistical analysis
Data were statistically analyzed using SPSS 17.0 (IBM SPSS Statistics). The normality of distribution was examined with the Shapiro-Wilk test. Experimental groups were compared using Mann-Whitney U test for the quantification of fluorescence intensity in whole brain imaging, brain slice imaging and tracer concentration of brain tissues and TN. The olfactory bulb was measured as a combined fluorescence quantification of both sides. Data with p < 0.05 were considered statistically significant
Results
Whole brain imaging analysis revealed that fluorescence was visible at the ventral view of the intact mouse brain and at the TN in IN and IN+US groups, but not in sham group ( Fig. 2 b). The low-intensity ultrasound facilitated NtoB delivery of dextran to the whole brain from the dorsal and ventral views at the 4-h mark post-euthanasia, but the fluorescence intensity decreased after a 24-h follow-up ( Fig. 2 b). The quantification of the fluorescence intensity from the whole-brain dorsal view revealed the delivery to the olfactory bulb significantly improved after US treatment (IN+US vs. IN-only: p = 0.0426; IN+US vs. sham: p = 0.0003) at 4 h; however, this facilitation was not observed in the whole-brain region analysis at 24 h ( Fig. 2 c). The fluorescence intensity from the whole brain ventral view demonstrated the tracer delivery to the olfactory bulb (IN+US vs. sham: p = 0.0005) and to the whole brain (IN+US vs. sham: p = 0. 0005; IN vs. sham: p = 0. 0005) at 4 h. The fluorescence of the TN was significantly higher at 4 h in the IN and IN+US groups than in the sham group (IN+US vs. sham: p = 0. 0003; IN vs. sham: p = 0. 0005).
In brain slice imaging, the fluorescence of 10-kDa dextran could be delivered to the OB, cerebral cortex, striatum, and brainstem in the IN+US group, but not in the IN-only group at the 4-h mark ( Fig. 3 a). The fluorescence of the NtoB tracer was not observed in the IN and IN+US groups at 24-h follow-up. The mean fluorescence intensities in specific regions including the olfactory bulb, cerebral cortex, brainstem, cerebellum, and TN, did note significantly differ between the IN and IN+US group at two follow-up time points ( Fig. 3 b). The fluorescence intensity of the TN at 4-h was higher than that at 24 h in the IN and IN+US groups (4 h vs. 24 h: p = 0. 0161 in IN group; 4 h vs. 24 h: p = 0. 0143 in IN+US group; Fig. 3 b).
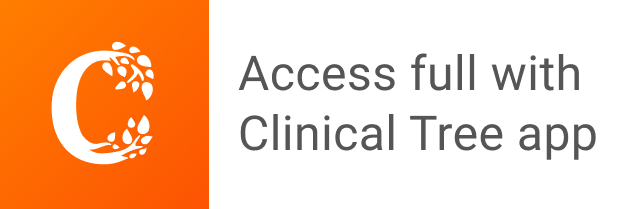