Introduction
Lung cancer is one of the most common malignancies, accounting for approximately 234,000 new cases and 160,000 deaths per year in the United States. Treatment for lung cancer depends on the disease stage at presentation, with disease at early stages treated by surgery or radiation alone and more advanced tumors receiving bi- or trimodality therapy.
Several studies have shown dosimetric benefits from particle therapy over intensity-modulated radiation therapy (IMRT) for some patients with lung cancer. This dosimetric superiority has been demonstrated for both early-stage and locally advanced disease and when three-dimensional (3D) conformal therapy and IMRT are compared with proton beam therapy (PBT). Notably, improvements in normal tissue dose have been noted mostly in low-dose regions, such as the volumes receiving 5 Gy (relative biological effectiveness [RBE]) (V5) or 10 Gy (RBE) (V10). This selective benefit results from the sharp dose buildup and abrupt dose drop beyond the target possible with PBT. This is particularly important for lung cancer, given the proximity of the lungs, heart, esophagus, major airways, large blood vessels, and spinal cord. The benefits in terms of reducing or eliminating the so-called low-dose bath with PBT cannot be accomplished at present with advanced photon techniques.
Clinical outcomes after particle therapy have been reported for both early-stage and locally advanced nonsmall cell lung cancer (NSCLC). The outcomes in these studies seem to be similar to or improved over those obtained with advanced photon modalities such as IMRT or volumetric modulated arc therapy. When this chapter was written, only one randomized trial that directly compared particle therapy with photons for locally advanced NSCLC had been reported. In that randomized phase II study, toxicity (pneumonitis) and local disease control were evaluated after patients received IMRT or passive scattered PBT, and no statistical differences in these end points were found between the two modalities. Future analyses from this study focus on comparisons of imaging data, blood samples, additional toxicity end points, and quality of life to assess how these factors may influence outcomes.
To summarize, proton therapy seems to have dosimetric benefits over various forms of photon therapy in some clinical situations, but thus far, no strong evidence has been found that “all comers,” that is, anyone with lung cancer, would benefit from PBT over advanced photon techniques. Thus, indications for PBT and criteria with which to select patients for PBT are of critical importance, particularly when passive scattering techniques are used.
Small cell lung cancer (SCLC) nearly always presents as locally advanced or metastatic disease, and therefore principles for PBT simulation, target delineation, and treatment planning are similar for locally advanced NSCLC and SCLC. However, experience with using PBT for SCLC is very limited. In one report of six patients with a median follow-up time of 12 months, 1-year overall survival (OS) and progression-free survival (PFS) rates were 83% and 66%, respectively. Thus although much of the discussion on NSCLC with regard to particle therapy techniques can be extrapolated to SCLC, additional investigation is needed regarding outcomes after PBT, including rates of local control and the benefit of such modalities as intensity-modulated proton therapy (IMPT).
Patient selection criteria
Passive scattering proton therapy
Appropriate criteria for identifying patients who may benefit from passive scattering PBT are important because in some cases, the treatment plans are better for advanced conformal techniques, such as IMRT, for the following reasons. First, passive scattering PBT involves some limitations in beam angles because of dose uncertainties in the beam path. Second, passive scattering PBT requires a “backstop” to provide much of the sharp dose falloff, which can be difficult in the context of early-stage lung parenchymal tumors. When no high-density tissue is present distal to the target, dose “spikes” can occur that substantially affect the dosimetry and contribute to more dose being delivered to the normal tissues than necessary, thereby leading to toxicity.
With these limitations in mind, from a dosimetric standpoint, the following patients are good candidates for passive scattering PBT rather than IMRT: (1) those with tumors located in tissue that can provide a suitable backstop to exploit the dose falloff properties of proton therapy, (2) those for whom IMRT is not feasible because of the inability to meet low dose constraints (e.g., V5, V10, or V20), and (3) those with anterior mediastinal tumors in that are proximal to the heart, lung, spinal cord, and esophagus.
Intensity-modulated proton therapy
IMPT offers the following benefits over passive scattering proton therapy: (1) improved conformality and (2) lesser influence of beam placement because the dose can be supplemented where necessary through “patching,” which also can reduce the magnitude of hotspots. IMPT can also be suitable for simultaneous integrated boost (SIB) regimens because, by placing the proton Bragg peak in the target, the target dose can be escalated while contributing very little dose to normal tissue. A comparative clinical trial is ongoing at MD Anderson to evaluate IMPT and IMRT for SIB.
Limitations of IMPT include (1) its greater sensitivity of dose to changes in anatomy and tumor size because of the lower number of beams used and its very high conformality, and (2) risk of reduced local control when spot scanning delivery interacts with respiratory motion, leading to targets being missed during certain phases of the respiratory cycle. Two specific scenarios in which dose to the lung cancer target has been reduced are in the development (or reduction of) atelectasis ( Fig. 18.1 ) and changes in tumor size during treatment.
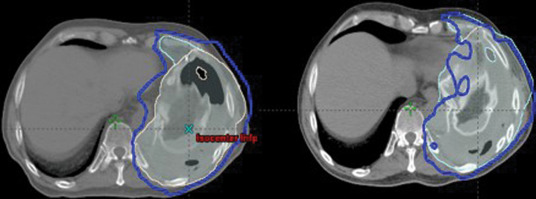
IMPT has often been used for (1) mediastinal but laterally displaced tumors, in which dose distribution is improved to the lung and esophagus; (2) extremely challenging cases in which dose constraints cannot be met with other techniques (e.g., large bilateral mediastinal masses); and (3) reirradiation, in which the goal is to almost completely avoid dose to one or more normal structures. As both the availability of and experience with IMPT increase, more patients have been selected for this approach, particularly those with locally advanced lung cancer. Several trials are ongoing to examine the safety and efficacy of IMPT for lung tumors, with particular attention being paid to local control given the concerns with respiratory motion interplay.
Treatment simulation
Treatment simulation should take place while patients have their arms over their heads; the selection of beam arrangement resembles that for photon techniques. Immobilization of the upper body should be used in conjunction with four-dimensional (4D) image acquisition to capture respiratory motion. If patients cannot raise their arms above their heads, the simulation can be done with their arms at their sides, although this setup can markedly limit the potential for dosimetric benefit, particularly if passive scattering PBT is being used.
Radiation dose and fractionation, target delineation, and treatment verification
A summary of these topics, discussed in further detail later, is provided in Table 18.1 .
Proton Beam Therapy | Locally Advanced Non-Small Cell Lung Cancer | Small Cell Lung Cancer | |
Prescribed dose and number of fractions | Many 1–10 fractionation schedules in use. At MD Anderson, peripheral disease is treated with 12.5 Gy (RBE) × 4 fractions, and central disease with 7 Gy (RBE) × 10 fractions | Standard regimen: 60 Gy (RBE) in 30 once-daily fractions with concurrent chemotherapy | Standard regimen: 45 Gy (RBE) in 30 twice-daily fractions with concurrent chemotherapy |
iGTV to CTV margin | 0 cm | 0.6–0.8 cm | 0.6–0.8 cm |
CTV to PTV setup margin | 0.5 cm (GTV to PTV) if daily CT available. If not available, 0.5–1.0 cm, ideally with fiducial placement | 1.0–1.5 cm without daily IGRT 0.5 cm with daily kV imaging 0.3 cm with daily CBCT | 1.0–1.5 cm without daily IGRT 0.5 cm with daily kV imaging 0.3 cm with daily CBCT |
Daily treatment verification | CT scanning (e.g., CBCT, CT-on-rails) if available and strongly recommended. If not available, strongly consider fiducial placement and then daily kV imaging with 0.5- to 1.0-cm setup margin | Daily kV imaging, weekly CBCT if available. | Twice-daily kV imaging (with each fraction), weekly CBCT if available |
Verification simulation | At least once during treatment (week 3–4), more often if significant tumor changes are observed | Consider after first week of treatment for bulky disease |
Radiation dose and fractionation
Several 1- to 10-fraction dose regimens are used for PBT. At MD Anderson, all proton prescription doses are reported with a constant RBE value of 1.1; we routinely use a dose of 50 Gy in 4 fractions for peripheral disease and 70 Gy (RBE) in 10 fractions for central disease. For locally advanced NSCLC that is to be treated with chemotherapy and radiation, the standard dose is 60 Gy (RBE) in 30 fractions, based on the recently published Radiation Therapy Oncology Group (RTOG) 0617 trial demonstrating no benefit from dose escalation to 74 Gy. For SCLC, the standard dose regimen remains 45 Gy (RBE) in 30 fractions delivered twice daily, based on the results of a randomized trial comparing once-daily to twice-daily radiation. However, the recently published phase III randomized Concurrent ONce-daily VErsus twice-daily RadioTherapy (CONVERT) study, which enrolled 547 patients, demonstrated equivalence in a cohort of patients with limited-stage SCLC when comparing 45 Gy in 30 fractions to 66 Gy in 33 fractions. The authors note that because the trial was designed to demonstrate the superiority of once-daily radiation and wasn’t powered to show equivalence, twice-daily regimens should continue to be considered the standard of care for such patients.
SIB regimens have also been used in the treatment of lung cancer with either photons or protons, and numerous studies are ongoing to evaluate the safety and efficacy of this approach.
Target delineation
Involved-field techniques with 4D computed tomography (CT)–based planning are used for both node-negative and node-positive disease, regardless of whether the treatment technique involves photons or protons. The gross tumor volume (GTV) is contoured on a CT scan of the chest with contrast with positron emission tomography scans used for guidance; histologic findings from mediastinoscopy or endobronchial ultrasonography are also used when available.
Two potential approaches can be used to expand the GTV to capture both internal motion and microscopic disease. The first involves expanding the GTV to the clinical target volume (CTV), followed by a further expansion to the internal target volume (ITV) to account for internal motion, followed by an expansion to the planning target volume (PTV) to cover daily variations in patient position and movement. The second approach, often used at MD Anderson, is to delineate the GTV, assess its internal motion, and create an “envelope” structure called the iGTV, which is then expanded to create the iCTV (which is very similar to the ITV). The advantage of the latter approach is its assessment of the internal motion of gross disease, which may be easier to delineate.
For early-stage lung cancer, per RTOG standards, no distinct CTV margin is included, and only a PTV is delineated. For locally advanced disease, standard GTV-to-CTV treatment margins (from the GTV [or iGTV] to the CTV) are 0.6 to 0.8 cm to cover microscopic disease, as defined in prior pathologic studies.
With regard to expansion to a PTV for proton therapy, there is no standard uniform PTV akin to that used in photon planning to cover errors in patient setup (typically fixed at 0.5–1.0 cm). Rather, the PTV for proton therapy includes two components: (1) the setup margin , which accounts for day-to-day setup errors and depends on the image-guided radiation therapy (IGRT) method used, and (2) the dosimetric margin , which is field-specific and encompasses proximal, distal, and lateral margins for that particular field (to account for dose uncertainty in the beam path).
The CTV-to-PTV setup margin for PBT corresponds to the 0.5 cm used for photon techniques and is an extension directly from the GTV. However, this setup margin presumes that cone-beam CT (CBCT) is available for daily localization. If daily CT imaging is not available, we strongly recommend considering fiducial placement, with daily kV imaging during treatment and a 0.5- to 1.0-cm PTV setup margin.
For locally advanced lung cancer, the following PTV margins are used: 1.0 to 1.5 cm without daily IGRT (e.g., kV imaging or CBCT); 0.5–1.0 cm for either 4D CT planning or CBCT, but not both; 0.5 cm for 4D CT planning and daily kV imaging; or 0.3 cm for 4D CT planning and CBCT guidance.
The field-specific dosimetric margin depends on the water-equivalent range relative to the most proximal and distal points of the CTV from a specific beam angle; these margins typically range from approximately 0.5 to 1.0 cm.
As an alternative to a geometric PTV, physicists at MD Anderson are exploring the use of beam-specific PTVs for passive scatter proton therapy planned with single-field optimization. This method provides significant advantages over the conventional method using a single PTV for all beams because the magnitude of each margin can be individualized for each field to account for tissue misalignment (patient setup error) and tissue heterogeneity without the use of a physical compensator.
Treatment verification
For daily treatment verification, most patients undergo daily kV imaging and at least one verification simulation to ensure that no substantial changes have taken place in tumor volume or other aspects of patient anatomy that would warrant repeat treatment planning. Use of midtreatment verification is particularly important for particle therapy, where seemingly minor changes in these factors can have pronounced dosimetric effects. If in-room CT capability is present, we recommend weekly CBCT scans in addition to the midtreatment verification scan.
Dose constraints
PBT involves several dose constraints, based in part on the number of fractions being delivered. These dose constraints are derived from those for photon therapy established in the National Comprehensive Cancer Network guidelines ( www.nccn.org ). Our institutional dose constraints for standard-fractionation, once-daily proton therapy are given in Table 18.2 . For twice-daily regimens, such as those given for SCLC, constraints are similar except for the dose to the spinal cord, which should be limited to a maximum dose of less than 40 Gy (RBE).
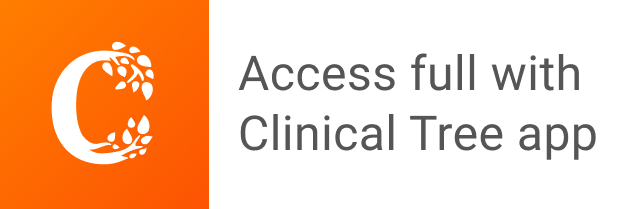