Introduction
The use of proton therapy for the treatment of cancer was first proposed by Robert Wilson in 1946. A beam of protons gives most of its dose at a fixed depth in the tissue known as the Bragg peak, with very little dose deposited beyond that depth. As a result of this physical characteristic, protons are better able to deliver high doses of radiation therapy with less scatter dose to nearby critical structures. Because of this perceived advantage, the use of proton beam radiation therapy has increased significantly in the past decade. Currently, 68 proton centers are in operation around the world, with 27 of them located in the United States.
Several studies have demonstrated the importance of dose escalation in the treatment of prostate cancer, with a higher radiation dose to the prostate leading to improved biochemical relapse-free survival and freedom from clinical failure rates. However, higher doses mean a higher risk of side effects. Because patients with prostate cancer can often expect to live for long periods after diagnosis and treatment, it is crucial that any treatment minimize the risk of side effects and negative effects on quality of life (QoL) for patients undergoing that treatment. As mentioned, the physical characteristics of proton beam therapy allow dose escalation to the tumor with less scatter and exit dose to the surrounding normal tissues, which, for prostate cancer, include the rectum and bladder.
This chapter summarizes the physics of proton therapy and recent technical advances in the delivery of proton beams, reviews clinical results, and describes the treatment protocol used at our institution for the treatment of prostate cancer.
Physics of proton beam radiation therapy
The proton is a positively charged particle; therefore, it has a more limited range in matter than x-rays. A beam made up of protons deposits most of its energy at a fixed depth known as the Bragg peak, with very little dose deposited beyond that depth ( Fig. 12.1 ). In contrast, an x-ray beam deposits its maximum dose just inside the patient’s body and then continues to travel through the body, depositing dose until it exits the body. The depth of the Bragg peak is based on the energy of the proton beam, with greater depths of deposition having higher doses. Normally, the Bragg peak is too narrow to treat an entire tumor. Therefore the width of the Bragg peak is spread out to cover the entire target volume with margin. This is known as the spread-out Bragg peak.
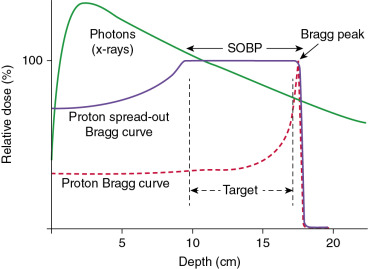
For patients with prostate cancer treated with protons, the most common beam arrangement is two beams coming in from the right lateral and left lateral directions. In contrast, x-rays with intensity-modulated radiation therapy (IMRT) use multiple angles or arcs to concentrate the radiation dose to the prostate, which results in low-dose scatter to the rest of the pelvis, including the bladder and rectum, with IMRT. Using proton therapy significantly reduces this scatter dose ( Fig. 12.2 ).
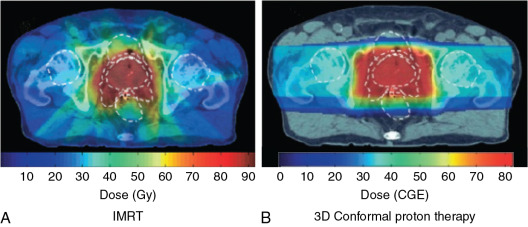
Modes of proton delivery
Currently, there are two types of proton therapy delivery: passively scattered proton therapy and pencil beam proton therapy. Passively scattered proton therapy consists of a monoenergetic beam of protons, which is then passed through a scattering system to create a larger beam of protons. At MD Anderson, the passively scattered beam used to treat patients comes in three sizes: small (10 × 10 cm), medium (18 × 18 cm), and large (25 × 25 cm). As mentioned before, the Bragg peak is usually spread out by using a range modulator wheel to cover the entire tumor with the margin in the direction of the beam. This beam is then further shaped by using two additional custom devices: a brass aperture and an acrylic tissue compensator. The aperture determines the shape of the radiation field (akin to multileaf collimators for x-rays), and the compensator shapes the distal edge of the proton beam dose to the shape of the target volume ( Fig. 12.3 ). These devices are created for each patient by milling machines located on-site at the Proton Therapy Center.
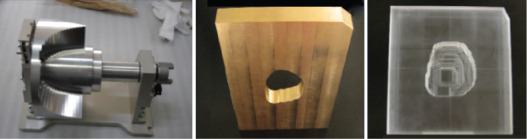
Pencil beam proton therapy (also known as active scanning proton therapy or spot scanning proton therapy) uses a narrow proton beam (“pencil beam”) to deliver dose by sequentially layering multiple pristine Bragg peaks (or “spots”) over the target volume. An electromagnetic field is used in the pencil beam to scan the protons in both directions perpendicular to the beam direction (i.e., x – and y -axes) without the need for a scattering device or an aperture. The depth of dose deposition (i.e., z -axis) is controlled by changing the energy of the protons in the pencil beam and delivering multiple layers of dose. Because this layering of dose allows better conformity of the proximal edge of the target volume compared with passively scattered proton therapy, normal tissue sparing is improved.
Pencil beam proton therapy has several other advantages over passively scattered proton beam therapy. Pencil beams therapy allows improved conformality of the proton dose, with improved tumor coverage and normal tissue sparing, especially around curved structures. The pencil beam does not require the use of brass apertures and acrylic compensators, which saves the time and effort normally needed to manufacture such hardware. It also makes it easier and faster for radiation therapists to treat the patient because they no longer need to mount the hardware on the treatment machine. Each beam angle with the passively scattered proton therapy needs its own set of aperture and compensator, therefore the number of beam angles that can be used per patient is limited. Pencil beams have less of a limit on how many beam angles can be used for treatment. Furthermore, the hardware in the pathway of the passively scattered proton therapy (which includes the range modulator wheel, aperture, and tissue compensator) leads to increased neutron production. As a consequence, pencil beam proton therapy also has the advantage of lower neutron doses, which should decrease the risk of secondary cancers and decrease the potential effect on implanted medical devices. Finally, pencil beams are required for intensity-modulated proton therapy (IMPT) with multifield optimization. IMPT allows further improvements in dose conformality and simultaneous integrated boosts to the gross tumor. However, pencil beam proton therapy requires more advanced planning and rigorous quality assurance to make sure that the dose delivered matches the treatment plan.
Fig. 12.4 illustrates a comparison of a pencil beam plan and a passively scattered plan for the treatment of a patient with prostate cancer. As can be seen, the radiation dose lateral and posterior to the prostate is much more conformal with the pencil beam. Most proton therapy centers now have pencil beams available for treatment. In fact, the newest proton therapy centers are being built with only pencil beam capability (and will no longer be able to treat with passively scattered proton beams).
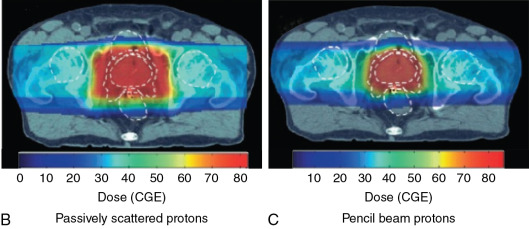
Clinical outcomes
Numerous studies have shown the benefit of dose escalation in the treatment of prostate cancer. Kuban et al. randomized 301 patients with stage T1b to T3 prostate cancer to either 70 or 78 Gy delivered with x-ray therapy. At a median follow-up time of 8.7 years, the patients who received 78 Gy had better rates of freedom from biochemical or clinical failure (78%) than the 59% for patients who received 70 Gy ( P = .004). The clinical failure-free survival rate in the 78-Gy arm was better than that in the 70-Gy arm (93% vs. 85%; P = .014), but no overall survival benefit was noted. Unfortunately, the 78-Gy dose was also associated with higher toxicity, with the rate of Radiation Therapy Oncology Group (RTOG) grade 2 or higher gastrointestinal (GI) toxicity being 26% versus 13% ( P = .013). No significant difference was found between groups in RTOG grade 2 or higher genitourinary (GU) toxicity (13% vs. 8%).
The Dutch Multicenter Trial randomized 669 patients with localized prostate cancer to either 68 or 78 Gy delivered with x-ray therapy. After a median follow-up time of 70 months, the 7-year freedom from failure rate was better in the 78-Gy arm than in the 68-Gy arm (54% vs. 47%; P = .04). Once again, late grade 2 or higher GI toxicity was higher in the 78-Gy arm than in the 68-Gy arm (35% vs. 25%; P = .04), but no significant difference was noted in late grade 2 or higher GU toxicity (40% vs. 41%; P = .6).
The Proton Radiation Oncology Group (PROG) 95-09 study randomized a total of 393 patients with stage T1b–T2b with prostate-specific antigen (PSA) levels less than 15 ng/mL treated at either Loma Linda University Medical Center (LLUMC) or Massachusetts General Hospital (MGH). All patients received three-dimensional conformal x-ray therapy to the prostate and seminal vesicles to a dose of 50.4 Gy, followed by a proton boost of either 28.8 cobalt Gy equivalent (CGE) or 19.8 CGE. Therefore the patients were randomized to either 79.2 CGE or 70.2 CGE. At LLUMC, patients were treated while supine with opposed lateral 250-MeV proton beams. At MGH, patients were treated in the lithotomy position with a single transperineal 160-MeV proton beam. At a median follow-up time of 8.9 years, patients who received 79.2 CGE were significantly less likely to have local failure, with a hazard ratio of 0.57. The 10-year biochemical failure rates according to the American Society of Therapeutic Radiation Oncology definition were 16.7% and 32.4% for the 79.2 CGE and 70.2 CGE arms, respectively ( P ≤ .0001). Patient-reported outcomes using the Prostate Cancer Symptom Indices and Distress Scales were published separately. At a median of 9.4 years, no difference in urinary obstruction/irritation ( P = .36), urinary incontinence ( P = .99), bowel problems ( P = .70), or sexual dysfunction ( P = .65) were noted between dose groups. Unfortunately, PROG 95-09 was not a randomized comparison of protons and x-rays; all of the patients received a combination of photons and x-rays. However, no increased GI toxicity was seen with dose escalation in this study when proton therapy was used for boost dosing.
Several single-institution reports have been published on outcomes after proton therapy for prostate cancer. Slater et al. published the LLUMC experience of 1255 patients treated between October 1991 and December 1997. Patients with 15% or greater risk of having pelvic lymph node metastasis according to the Partin tables were treated with a conformal “boost” with protons to a dose of 30 CGE in 15 fractions given to the prostate and seminal vesicles, followed by a conformal treatment with x-rays to a dose of 45 Gy to the prostate, seminal vesicles and the first- and second-echelon lymphatics. Patients who did not have this risk were treated with proton therapy only to a total dose of 74 CGE in 2-CGE fractions. These patients were treated with a rectal balloon placed daily, usually with one field per day. With a median follow-up time of 62 months, the overall biochemical disease-free survival rate was 73% and depended on both the initial PSA and the PSA nadir reached after the treatment. In patients with an initial PSA of level 4.0 ng/mL or less, the biochemical disease-free survival rate was 90%, and in patients with posttreatment PSA of 0.5 ng/mL or less, 87%. The actuarial 5-year and 10-year rates of freedom from grade 3 and 4 GI toxicity were both 99%. The actuarial 5-year and 10-year rates for freedom from grade 3 and 4 GU toxicity were also both 99%.
Bryant et al. published the outcomes of 1327 patients treated with protons at the University of Florida Jacksonville between 2006 and 2010. With a median follow-up time of 5.5 years, the 5-year freedom from biochemical progression rates were 99%, 94%, and 74% in low-risk, intermediate-risk, and high-risk patients, respectively. The actuarial 5-year rates of late grade 3 or higher on the Common Terminology Criteria for Adverse Events, version 4.0 (CTCAE 4.0) for GI and GU toxicity were 0.6% and 2.9%, respectively. No significant changes were noted in median and mean Expanded Prostate Cancer Index (EPIC) summary scores for the bowel, urinary irritative/obstructive, and urinary incontinence domains. Only sexual function summary scores declined from baseline to 5 years in patients who did not receive hormone ablation therapy.
Pugh et al. reported patient-reported outcomes of 291 patients after proton therapy at MD Anderson Cancer Center who had a minimum follow-up of 2 years. All patients were treated with opposed lateral beams to a total dose of 76 CGE in 2-CGE fractions. Interestingly, 226 patients were treated with passively scattered proton therapy, and 65 patients were treated with pencil beam proton therapy. Cumulative rates of grade of 2 or higher GU and GI toxicity at 24 months were 13.4% and 9.6%, respectively. One patient had grade 3 GI toxicity, but none had grade of 4 (or higher) toxicity. No patients had grade 3 (or higher) GU toxicity. Patients receiving passively scattered proton therapy had a slightly higher rate of argon plasma coagulation compared with pencil beam proton therapy, but that apparent difference was not statistically significant (4.4% vs. 1.5%; P = .21).
Several retrospective comparisons of protons and IMRT have been made. A Surveillance, Epidemiology, and End Results (SEER)-Medicare analysis by Sheets et al. showed a lower rate of GI morbidity (relative risk [RR]: 0.66) with IMRT than with protons in a propensity score–matched comparison. However, this analysis was severely limited, as any GI procedure performed after the treatment was coded as a morbidity. Hoppe et al. compared patients undergoing either proton therapy or IMRT by using prospectively collected QoL data from the EPIC; 1243 proton patients were treated with 76 to 82 CGE and 204 IMRT patients were treated with 75.6 to 79.4 Gy. No difference was seen between the two groups for the bowel, urinary incontinence, urinary irritative/obstructive, and sexual domains. However, more patients in the IMRT group reported moderate/big problems with rectal urgency ( P = .02) and frequent bowel movements ( P = .05) compared with patients in the proton therapy group. Fang et al. compared 181 proton therapy patients and 213 IMRT patients treated between 2010 and 2012 in terms of maximum acute and late GI/GU CTCAE-graded toxicities. On multivariate analysis, no statistically significant differences were found in the rates of acute/late grade 2 GU (or higher) and GI toxicities between the two groups. Yu et al. analyzed a Medicare database of 27,647 men and found that patients who received proton therapy had significant less GU toxicity at 6 months compared with IMRT (5.9% vs. 9.5%; P = .03); however, the difference disappeared by 12 months (18.8% vs. 17.5%; P = .66). No difference was found in GI or other toxicity at 6 or 12 months.
Proton therapy may also reduce the risk of secondary cancer when compared with IMRT. Several analyses have predicted that IMRT would increase the risk of secondary cancer, whereas proton therapy would decrease the risk. A retrospective matched cohort analysis of 558 proton patients and 558 x-ray patients in the SEER registry published by Chung et al. showed that proton therapy was associated with a significant reduction of secondary cancer risk (RR: 0.52; P = .009) relative to x-ray therapy. However, the limitations of this retrospective study led the authors to state that these results should be considered as hypothesis-generating.
These results show that proton therapy is an effective and safe method for the treatment of prostate cancer. However, because no randomized trial has directly compared proton therapy with IMRT, it is difficult to know if protons are superior to IMRT in terms of efficacy or risk of side effects. One randomized trial is currently ongoing, the Proton Therapy versus IMRT for Low or Intermediate Risk Prostate Cancer (PARTIQoL). However, we will need to wait several years until the results of that trial are available.
Proton treatment at MD Anderson Cancer Center
Patient selection
Although the results of the PARTIQoL trial are not yet available, there are probably several specific instances where proton therapy may be especially beneficial. For instance, we recommend proton therapy for younger patients (although the definition of “younger” can vary significantly among clinicians) and for patients with larger prostates, especially a large medial lobe. Trying to cover a large medial lobe with IMRT usually leads to irradiating a much larger area of the bladder than with proton therapy. However, we routinely recommend that men with a very large medial lobe consider having that lobe surgically removed via a transurethral resection of the prostate or greenlight laser enucleation before radiation therapy. If a patient were to undergo such a procedure, we recommend waiting for 2 to 3 months before starting radiation therapy to allow adequate healing to minimize the risk of urinary incontinence after the radiation therapy.
As is true for IMRT, we do not recommend proton therapy for patients with certain collagen vascular diseases (such as lupus and scleroderma) or inflammatory bowel disease (i.e., Crohn disease and ulcerative colitis). We also do not treat patients with metal in the pelvis (most often artificial hips) in the pathway of the proton beam, for two reasons. The first reason is that the metal can significantly block the proton beam, which could decrease the dose delivered to the prostate. The second reason is that artifacts from the metal make treatment planning less accurate because of increased uncertainty in tissue density calculation from the planning computed tomography (CT) scan. We also discourage patients who are pacemaker dependent from undergoing proton therapy because of the neutron dose from the protons and their potential effects on the pacemaker. When we do treat patients with pacemakers (or any other implanted electronics), we treat them with the pencil beam to try to minimize the neutron dose as much as possible.
Fiducial placement
Once a patient is deemed to be a candidate for proton therapy as the definitive treatment for prostate cancer, fiducial placement and treatment simulation are planned.
At MD Anderson, the fiducials are inserted transrectally under ultrasound guidance by either the radiation oncology or urology teams. However, at other institutions, fiducials may be placed by interventional radiologists. Fiducial placement may be more important for proton therapy than for IMRT because of the risk of shadowing of the proton dose from the fiducials. Tables 12.1 and 12.2 show the actual amounts of dose attenuation from various types of fiducials. Of the three types of fiducials listed, gold fiducials have the highest proton attenuation, and so we use the carbon/ZrO 2 fiducial at ( Fig. 12.5 ) at MD Anderson. We usually place two fiducials in the prostate (as opposed to three for IMRT) to minimize the risk of dose shadowing in tumor areas. We also try to place the fiducials in areas where little or no cancer is present.
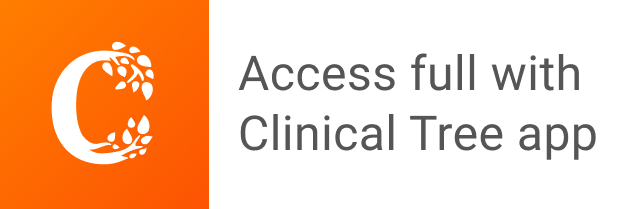