Remote-controlled and teleoperated robotic systems mark transformative advancements in interventional radiology (IR), with the potential to enhance precision, reduce radiation exposure, and expand access to care. By integrating robotic devices with imaging guidance, these systems enable precise instrument placement and navigation, thereby improving the efficacy and safety of minimally invasive procedures. Remote-controlled and teleoperated robotic systems—operated by clinicians using control interfaces from within or adjacent to the procedure room—are being adopted for both percutaneous and endovascular interventions. In contrast, although their application is still experimental, teleoperation over long distances hold promise for extending IR services to medically underserved areas by enabling remote procedures. This review details the definitions and components of remote-controlled and teleoperated robotic systems in IR, examines their clinical applications in percutaneous and endovascular interventions, and discusses relevant challenges and future directions for their incorporation into IR practices.
Introduction
Interventional radiology (IR) is a medical specialty that uses imaging-guided, minimally invasive procedures for the diagnosis and treatment of medical conditions. IR procedures have been demonstrated to minimize adverse events, reduce health care costs, and provide valuable services in coordination with other medical specialties. However, their success is highly dependent on the clinician’s experience and underlying skillset especially given the intricate nature of imaging-guided procedures.
The integration of robotic systems into IR, therefore, represents a significant technological opportunity that may help address such limitations. While the adoption of robotics into IR has been slower when compared to surgical specialties, there is growing interest in incorporating robotic systems due to their potential to enhance technical precision, standardize procedures, and reduce radiation exposure to healthcare workers. Moving beyond manual instrument control, remote-controlled and teleoperated robotic systems are now emerging as innovative tools with the potential to improve procedural outcomes and expand access to IR.
This literature review provides a comprehensive overview of remote-controlled and teleoperated robotic systems in interventional radiology. We will outline definitions of remote-controlled and teleoperated systems, describe the components that build these systems, and explore their current use in percutaneous and endovascular interventions. We will present relevant examples of how remote-controlled robotic systems have been employed in specific procedures and discuss the current state of teleoperated robotic systems. Finally, we will address the challenges of implementing teleoperated robotic systems in IR and suggest future directions for their integration into clinical practice.
Remote-controlled and teleoperated robotic systems
Definition of robotic systems and key terminology
In the context of interventional radiology, robotic systems can be defined as computerized platforms that coordinate motorized robotic manipulators—often integrated with navigation systems—to semi-autonomously or autonomously assist in minimally invasive procedures. , Remote-controlled and teleoperated robotic systems are operated by clinicians from within the procedure room or an adjacent control area, using a control console to manipulate the robotic device. While still experimental, long-distance teleoperated systems may enable clinicians to operate the robotic device across significant geographical distances (e.g., transcontinental) via network connections.
Key terms in the development and evolution of robotic systems are haptic feedback and navigation systems, which respectively provide tactile and visual support during robotic-assisted interventions. Haptic feedback, which refers to tactile and kinesthetic sensations provided by the robotic device to the operator, is designed to simulate the sensory experience of manipulating instruments by hand. , Because interventionalists often rely on haptic feedback to confirm the success of key procedural steps and to modify their hand movements, the implementation of haptic feedback is crucial for ensuring the safety and efficacy of a robotic system—especially during highly tactile tasks such as needle placement, catheter guidance, and embolization.
Navigation systems can supplement the tactile and kinesthetic assistance provided by haptic feedback by further offering visual support. Navigation systems can be defined as computerized platforms used before or during robotic manipulator motion to facilitate instrument holding, trajectory planning, and real-time tracking. These systems integrate with cameras (“optical”), , electromagnetic transmitters (“electromagnetic”), , or real-time imaging modalities (“imaging-based”) , to enhance procedural accuracy.
Components of a robotic system
Robotic systems used in IR consist of several components: robotic manipulators, control interfaces, navigational systems, and software. Robotic manipulators, or arms, are motorized and reprogrammable mechanical devices designed to hold, move, or operate instruments like needles and catheters. These arms replicate movements of the clinician’s upper extremity while reducing tremors and instability, thus improving procedural precision and accuracy. As the keystone of the system, they effectively serve as a substitute for the clinician’s hand during interventions.
However, the robotic manipulators require a method with which the operator can control them. The control interface of a robotic system, therefore, serves as the nexus for the planning, guiding, and manipulation of the robotic arm. These units often feature apparatus such as joysticks, touchscreens, or specialized control consoles with haptic feedback, offering an intuitive method for clinicians to operate the robotic system. In remote-controlled systems, the control unit is located near the patient, within or adjacent to the procedure room. In long-distance teleoperated systems, however, the control units are placed at distant locations, enabling clinicians to control the robotic device remotely via network connections.
As discussed earlier, navigational systems are crucial for tracking, locating, and guiding the robotic arm in 3-dimensional space. Depending on manufacturer and whether it is intended for use inside or outside the gantry of imaging scanners, various navigation methods—optical, electromagnetic, or real-time imaging—may be employed to assist with arm positioning.
Finally, the robotic system software integrates all these components, coordinating the robotic arm’s movement with imaging to optimize the robotic procedure workflow ( Fig. 1 ). It provides the control unit’s user interface, supports trajectory calculation, , and implements safety features like motion constraints and collision avoidance algorithms. , In long-distance teleoperated systems, the software may also rely on stable network connections and supporting network infrastructure to reduce latency and maintain accuracy during remote procedures. ,
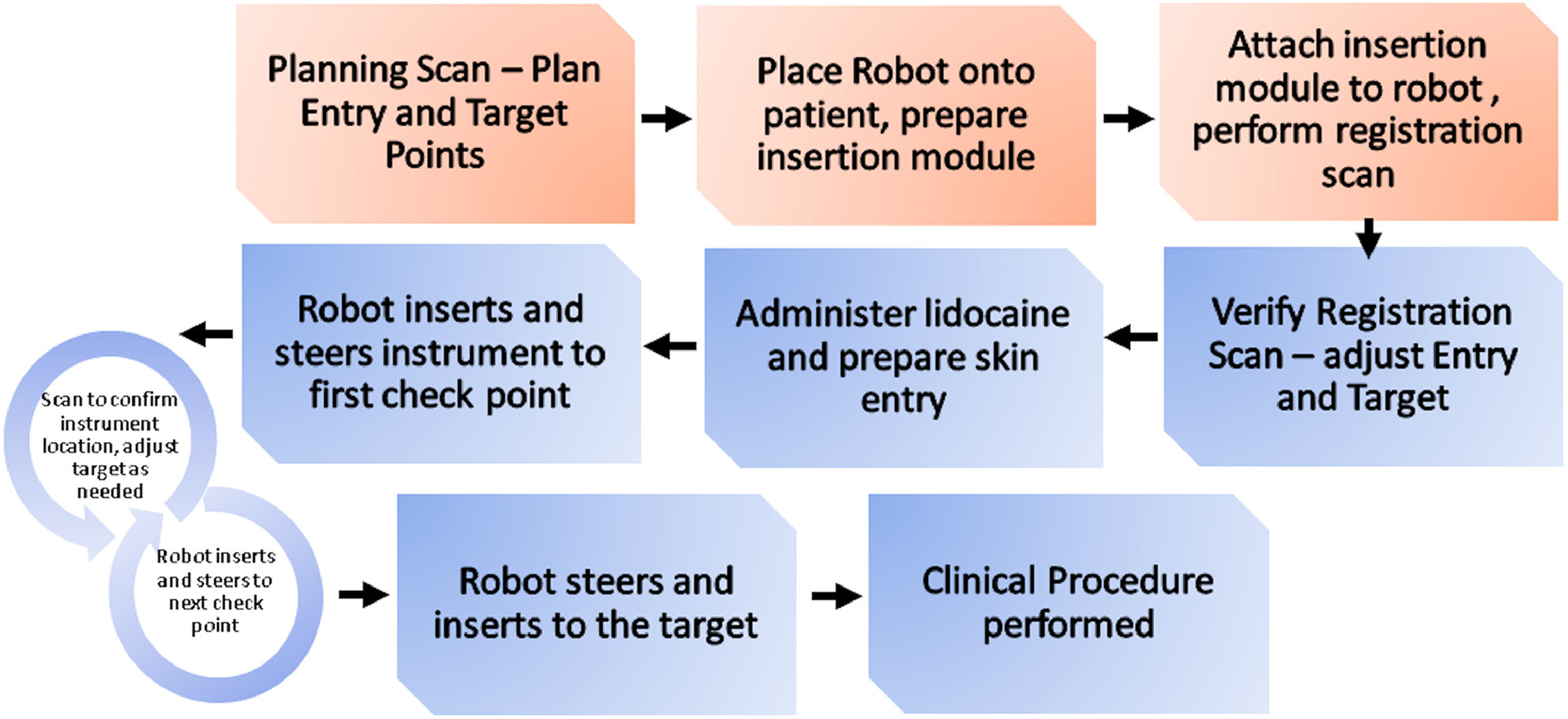
Clinical applications in percutaneous and endovascular interventions
Remote-controlled and teleoperated robotic systems have been developed for use in both percutaneous and endovascular interventions. In the context of percutaneous procedures, robotic systems have traditionally concentrated on needle manipulation, with an emphasis on needle driving and placement during biopsies and tumor ablations. In contrast, endovascular robotics have primarily focused on robotic catheters, which can be steered and guided accurately to an endovascular target.
To our knowledge, the majority of prior reviews on robotic systems have focused on and organized their article by specific robotic manufacturer and platform, rather than on the procedures with which such systems have been used. , To complement the existing body of knowledge, our review will instead focus on how remote-controlled and teleoperated robotic systems have been used in specific clinical procedures, organizing the use of robotic systems by procedure rather than by system.
Tables 1 , 2 , and 3 respectively summarize the use of robotic systems in percutaneous, endovascular, and long-distance teleoperated procedures, as reported in the literature.
Procedure | Device (Robot Name, Company Name, Country) | Literature Reference (Author, Year) | |
---|---|---|---|
Needle Biopsy | Lung Biopsy | ACE (XACT Robotics, Israel) | Alexander et al. (2024) ; Bodard et al. (2024) |
MAXIO (Perfint Healthcare, India) | Chu et al. (2014) ; Johnston et al. (2022) ; Bodard et al. (2024) | ||
ROBIO EX (Perfint Healthcare, India) | Bodard et al. (2024) | ||
Prostate Biopsy | iSR’obot MonaLisa (Biobot Surgical Ltd., Singapore) | Alargkof et al. (2024) | |
Remote Controlled Manipulator (Soteria Medical BV, Netherhands) | Barral et al. (2019) | ||
ROBIO EX (Perfint Healthcare, India) | Kumar et al. (2022) | ||
Breast Biopsy | Stormram 1-4 Series (University of Twente, Netherlands) | Groenhuis et al. (2018) | |
Sunram 5 (University of Twente, Netherlands) | Groenhuis et al. (2020) | ||
Liver Biopsy | MAXIO (Perfint Healthcare, India) | Bodard et al. (2023) | |
ROBIO EX (Perfint Healthcare, India) | |||
ACE (XACT Robotics, Israel) | |||
AcuBot (Johns Hopkins, USA) | |||
EPIONE (Quantum Surgical, France) | |||
Tumor Ablation | Liver, Renal, and Abdominal Tumor Ablation | Needle Placement System (DEMCON Advanced Mechatronics, Netherlands) | Heerink et al. (2019) |
EPIONE (Quantum Surgical, France) | de Baére et al. (2022) ; Bonnet et al. (2024) | ||
Osteoid Osteoma Ablation | TiRobot (TINAVI, China) | Li et al. (2024) | |
Lung Tumor Ablation | MAXIO (Perfint Healthcare, India) | Johnston et al. (2023) | |
Spinal Intervention | Spinal Cord Injections | Not commercially available | Squires et al. (2018) ; Squires et al. (2021) |
Procedure | Device (Robot Name, Company Name, Country) | Literature Reference (Author, Year) | |
---|---|---|---|
Coronary and Peripheral Vascular Intervention | Percutaneous Coronary Intervention (PCI) | CorPath 200 (Corindus Vascular Robotics, USA) | Weisz et al. (2013) |
CorPath GRX (Corindus Vascular Robotics, USA) | Smitson et al. (2018) ; Leung et al. (2024) ; Häner et al. (2023) ; Kagiyama et al. (2021) | ||
Peripheral Artery Disease (PAD) Angioplasty | CorPath 200 (Corindus Vascular Robotics, USA) | Mahmud et al. (2016) | |
CorPath GRX (Corindus Vascular Robotics, USA) | Mahmud et al. (2020) | ||
Carotid Artery Stenting | Magellan (Hansen Medical, USA) | Jones et al. (2021) | |
Aneurysm Repair, Embolization, and Foreign Body Retrieval | Endovascular Aortic Aneurysm Repair | Sensei X (Hansen Medical, USA) | de Ruiter et al. (2015) |
Magellan (Hansen Medical, USA) | Perera et al. (2017) | ||
Fenestrated Endovascular Aneurysm Repair (FEVAR) | Magellan (Hansen Medical, USA) | Riga et al. (2013) | |
Uterine Artery Embolization | Magellan (Hansen Medical, USA) | Rolls et al. (2014) | |
Prostatic Artery Embolization | Magellan (Hansen Medical, USA) | Bagla et al. (2017) | |
Aortic Pseudoaneurysm Coil Embolization | Magellan (Hansen Medical, USA) | Lu et al. (2016) | |
IVC Filter Retrieval | Magellan (Hansen Medical, USA) | Owji et al. (2017) | |
Pulmonary Artery Foreign Body Removal | Magellan (Hansen Medical, USA) | Wolujewicz et al. (2016) | |
Cardiac Ablation | Atrial Fibrillation Ablation | Sensei X (Hansen Medical, USA) | Lorgat et al. (2012) ; Thomas et al. (2012) |
Niobe Robotic Magnetic Navigation System (Stereotaxis Inc., USA) | Maurer et al. (2017) ; Jin et al. (2016) ; Virk et al. (2019) | ||
Atrioventricular Nodal Reentrant Tachycardia (AVNRT) Ablation | Niobe Robotic Magnetic Navigation System (Stereotaxis Inc., USA) | Reents et al. (2017) | |
Atrial Tachycardia Ablation | Niobe Robotic Magnetic Navigation System (Stereotaxis Inc., USA) | Mehta et al. (2008) | |
Ventricular Tachycardia Ablation | Niobe Robotic Magnetic Navigation System (Stereotaxis Inc., USA) | Di Biase et al. (2017) | |
Neurovascular Intervention | Basilar Artery Aneurysm Coiling | CorPath GRX (Corindus Vascular Robotics, USA) | Mendes Pereira et al. (2020) |
Cerebral Aneurysm Coiling | CorPath GRX (Corindus Vascular Robotics, USA) | Mendes Pereira et al. (2024) | |
Diagnostic Angiography and Carotid Artery Stenting | CorPath GRX (Corindus Vascular Robotics, USA) | Sajja et al. (2020) ; Nogueira et al. (2020) ; Weinberg et al. (2020) ; Costa et al. (2023) | |
Endovascular Thrombectomy | CorPath GRX (Corindus Vascular Robotics, USA) | Kaneto et al. (2023) ; Tomasello et al. (2024) | |
Cerebral Angiography and Robotically Assisted Intracranial Interventions (RAII) | Magellan (Hansen Medical, USA) | Vuong et al. (2017) |
Literature Reference (Author, Year) | Procedure | Device (Robot Name, Company Name, Country) | Research Model | Distance Between Operator and Robotic Manipulator |
---|---|---|---|---|
Madder et al. (2017) | PCI | CorPath 200 (Corindus Vascular Robotics, USA) | Human | Isolated Rooms within Same Hospital |
Madder et al. (2018) | PCI | CorPath GRX (Corindus Vascular Robotics, USA) | Endovascular Simulator ( ex vivo ); animal | 7.5 kilometers ( ex vivo ); 166 kilometers (animal model) |
Madder et al. (2021) | PCI | CorPath GRX (Corindus Vascular Robotics, USA) | Endovascular Simulator ( ex vivo ) | Regional (206 miles); Transcontinental (3,085 miles) |
Patel et al. (2019) | PCI | CorPath GRX (Corindus Vascular Robotics, USA) | Human | 20 miles |
Yang et al. (2024) | Ultrasound Guidance for Percutaneous Puncture | MGIUS-R3 Robotic Ultrasound System (Wisonic Medical Technology Co., Ltd., China) | Phantom ( ex vivo ); animal | Isolated Rooms within Same Hospital |
Chai et al. (2024) | Diagnostic Ultrasound Examination | MGIUS-R3 Robotic Ultrasound System (Wisonic Medical Technology Co., Ltd., China) | Human | 35.9 kilometers |
Remote-controlled percutaneous robotics
Needle biopsy
The use of remote-controlled robotic systems in needle biopsies have been well-documented across various organ systems ( Table 1 ). , , Robotics for percutaneous needle placement can be largely categorized into needle-guiding robots—which plan the needle trajectory and align the needle accordingly— and needle-driving robots, which take the additional step of inserting the needle through the patient’s skin.
Lung biopsies
There has been increasing interest in the use of percutaneous robotic systems in CT-guided lung biopsies. While robotic bronchoscopy is another method of robot-assisted lung biopsy—used more typically by pulmonologists—it is often limited to more central lung lesions and associated with smaller collected tissue samples. In response to such limitations, there have been attempts to instead augment CT-guided lung biopsies with robotics in the context of IR.
For example, a recent retrospective cohort study of percutaneous lung biopsies by Alexander et al. demonstrated that the ACE robotic system (XACT Robotics, Caesarea, Israel)— a patient-mounted needle-driving robotic system ( Fig. 2 )—had comparable clinical outcomes to manual needle insertion, but with the added benefit of out-of-plane navigation. Similarly, needle-guiding robotic systems, such as the ROBIO EX and MAXIO robotic systems (Perfint healthcare, Chennai, India), have been used in a limited number of patients for CT-guided percutaneous lung biopsies in Hong Kong and London. Consistent with these studies, a systematic review by Bodard et al. of 4 studies involving both needle-guiding (i.e., ROBIO EX and MAXIO) and needle-driving (i.e., XACT ACE) robotic systems used for CT or PET/CT-guided lung biopsies demonstrated that the use of robotic systems was associated with fewer needle adjustments, shorter procedure time, and reduced patient radiation exposure when compared with manual biopsies.
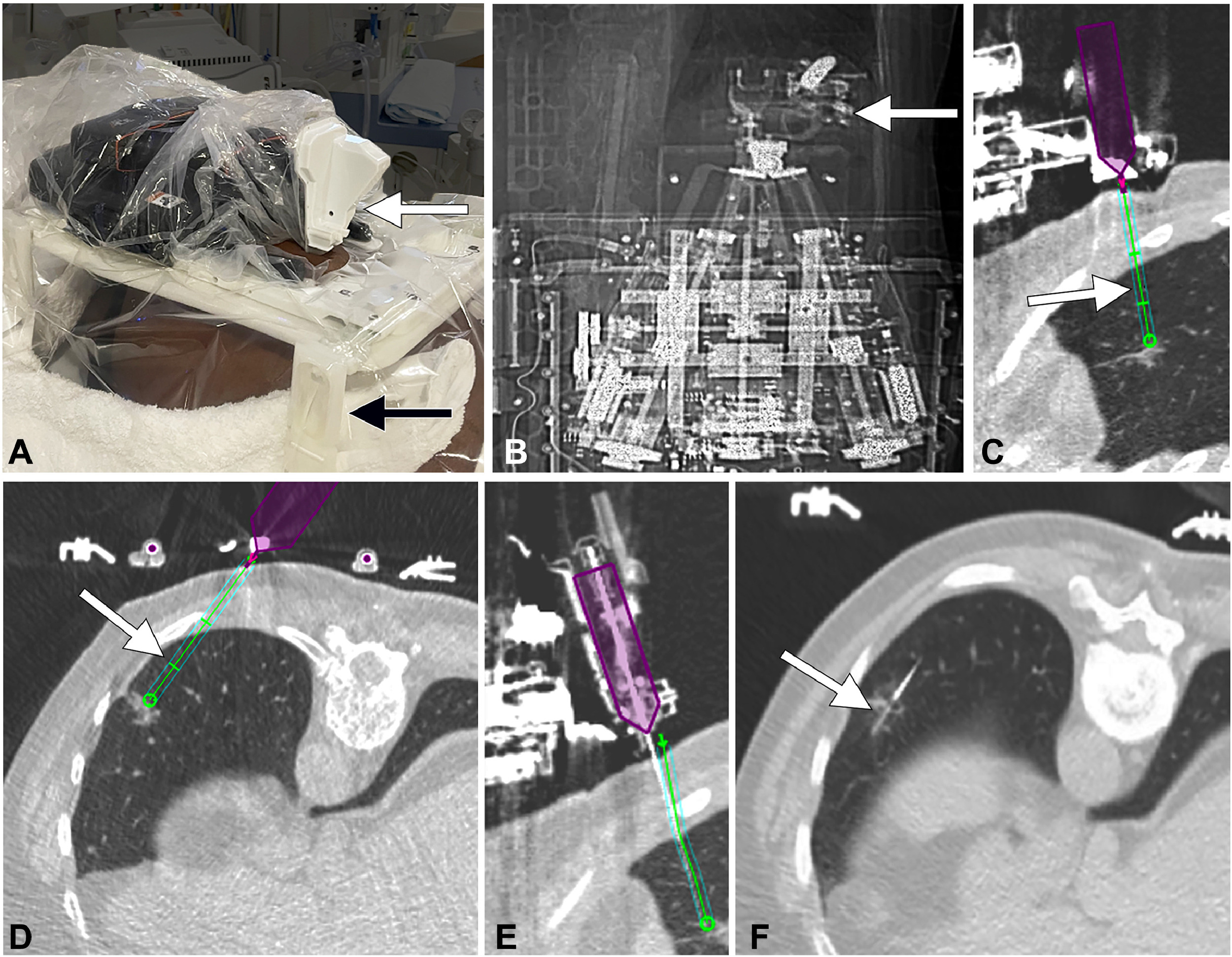
Prostate biopsies
While ultrasound (US)-guided prostate biopsies are commonly performed by urologists, the advent of magnetic resonance (MR) imaging-guided and image fusion-guided biopsies continue to expand the role of interventional radiologists in the screening, diagnosis, and intervention of prostate cancers. , Accompanying this trend, there has also been increasing interest in implementing robotic assistance, with the goals of improving precision and reducing interoperator variability.
The benefits of robotic systems in prostate biopsy are exemplified by a prospective observational study conducted by Alargkof et al., which suggests that robotic-assisted transperineal MRI/US fusion-guided prostate biopsy may be associated with a flattened operator learning curve as compared to without robotic assistance. Additionally, in an observational cohort study, Barral et al. demonstrated that in-bore transrectal MRI-guided biopsy with remote-controlled robotic assistance is feasible with high diagnostic yield, especially in cases where previous biopsies were inconclusive. Moreover, procedure times were shorter when compared to MRI-guided biopsies performed manually, again suggesting higher levels of procedural efficiency and precision. More recently, Kumar et al. demonstrated the feasibility of a PET/CT-guided prostate biopsy using the ROBIO EX robotic system (Perfint healthcare, Chennai, India) in a single-center clinical trial with 78 participants. The study demonstrated a high diagnostic yield (96% on first attempt) with only minor complications.
Breast biopsies
Robotic systems have also been investigated for MR and US-guided breast biopsies, with the aims of overcoming the high level of training and experience required on part of the physician. However, in contrast to the lung or prostate biopsies discussed previously, attempts have primarily focused on autonomic lesion identification or even fully autonomous biopsies, rather than master-slave robotic insertion of the biopsy needle. One example of a fully autonomous, MR-compatible, needle-driving breast biopsy robotic system was developed and evaluated by Groenhuis et al. (Stormram 1-4 series, Sunram 5). , While human studies have yet to be undertaken, the robotic system demonstrated high precision in breast phantoms. Similarly, Welleweerd et al. designed a robotic system for ultrasound-guided breast biopsies that provides real-time imaging of the target lesion, calculates the optimal needle trajectory, and provides a needle stop mechanism for when the clinician manually inserts the biopsy needle. However, to our knowledge, there has been no known study investigating the use of robot-assisted breast biopsies in humans.
Liver biopsies
The use of remote-controlled robotic systems in liver biopsies and tumor ablations have been well described by Bodard et al., who conducted a systematic review with 10 studies encompassing 429 percutaneous thermal ablations and 57 biopsies with needle-guiding and needle-driving robotic systems. The study concluded that the robotic assistance reduced the mean deviation of probes by an average of 30%, and consequently 40% fewer probe readjustments, thereby suggesting benefits in precision and efficiency. Moreover, the use of robotic systems was associated with a significant reduction in procedure time and radiation dose, for both the patient and the operator, compared to manual procedures.
Tumor ablation
In addition to facilitating needle biopsies, remote-controlled robotic systems have demonstrated potential benefits during tumor ablation procedures in terms of accuracy, procedural safety, and therapeutic outcomes ( Table 1 ).
Liver, renal, and abdominal tumor ablations
In CT-guided liver tumor ablations, robotic systems may enable accurate needle insertion along pre-planned trajectories—via needle guiding and/or driving—and thereby maximize procedural precision while minimizing complication risk. In a prospective randomized control trial, Heerink et al. reported that the use of the Needle Positioning System (DEMCON Advanced Mechatronics, Enschede, Netherlands) minimized the need for probe repositioning while demonstrating a higher accuracy for out-of-plane targets; however robotic assistance was associated with longer targeting times. Similarly, a pilot study with 24 patients using the EPIONE robotic system (Quantum Surgical, Montpellier, France) demonstrated the feasibility of needle-guiding robot-assisted liver biopsy (22/23 lesions), with 70.8% of tumors requiring no adjustment and without any adverse events during 6-month follow-up. More recently, there have been attempts to use a patient-mounted robotic system for MR-guided microwave ablation. However, such studies have only been conducted on phantoms thus far. In a recent retrospective analysis of 41 patients with abdominal tumors (23/41 liver; 14/41 kidney; 3/41 adrenal gland; 1/41 retroperitoneum), Bonnet et al. demonstrated that CT-guided percutaneous thermal ablation with the EPIONE needle system (Quantum Surgical, Montpellier, France) was potentially feasible, safe, and precise. Such findings suggest that the benefits of robotic assistance may apply beyond liver tumors, and could potentially be generalized to abdominal tumors as a whole.
Osteoid osteoma ablations
For the treatment of osteoid osteomas, which require precise needle placement for effective radiofrequency ablation (RFA), robotic systems may enhance accuracy. While studies investigating this topic have been limited, Li et al. examined the use of the TiRobot robotic system (TINAVI, Beijing, China) in percutaneous radiofrequency ablation of osteoid osteomas in a retrospective cohort study of 21 patients. In conjunction with a 3D C-arm, the system assists with trajectory planning and remote-controlled placement of biopsy and RFA needles into the tumor. The procedure was successfully completed in all patients with significant pain relief and without any complications.
Lung tumor ablations
Robotic systems have also been applied to the ablation of pulmonary tumors. In a 2023 comparative cohort study, Johnston et al. reported that CT-guided radiofrequency ablation of pulmonary metastases with the MAXIO robotic system (Perfint healthcare, Chennai, India) was associated with fewer adverse events and needle manipulations when compared to manual placement.
Spinal intervention
In spinal procedures such as vertebroplasty, kyphoplasty, and spinal biopsies, robotic systems could potentially assist in navigating instruments through the intricate anatomical structures of the vertebrae. While literature on this topic is limited, there have been attempts to develop a needle-guiding robotic system for spinal cord injections. , This system has been tested on phantoms and swine cadaver, but it has yet to be used in humans ( Table 1 ).
Challenges and future directions in percutaneous robotics
While the previously mentioned robotic systems have demonstrated potential feasibility for percutaneous interventions, several areas of research seek to further improve precision and safety. First, needles often do not contain actuated elements, and are instead steered via a manipulator outside the body. A specially shaped tip on the needle causes passive steering due to asymmetric tissue forces. Actively steerable needles, however, enable articulation of the needle’s tip while inside the body, potentially leading to an increased ability to avoid critical structures and blood vessels during insertion. Common actuation technologies include tendons, programmable bevel needles, and concentric tubes. Secoli et al. demonstrated the first in vivo deployment of such an actively steered needle, using a neurosurgical programmable bevel needle in an ovine model. However, the difficulty of miniaturizing such actuation technologies is still a significant limitation.
In addition, the absence of haptic feedback in commercial systems is notable as needle steering is a highly contact-rich task. Several studies have demonstrated the potential utility of providing haptic feedback, most often through commercial haptic interfaces. Aggravi et al. implemented kinesthetic and tactile feedback to convey cutting and friction forces under ultrasound guidance and demonstrated significantly higher targeting accuracy in an in vitro gelatin phantom. Providing multi-modal haptic feedback has been shown to improve needle steering performance in several similar studies. , One potential limitation to these approaches is that the kinesthetic feedback provided by commercial interfaces often can interfere with the stability of operation, especially in the presence of any communication delays between the control unit and robotic manipulator. To address this, Meli et al. implemented a novel haptic feedback scheme that ensured stable driving of the needle and found improved needle stiffness discrimination and penetration depth accuracy. The implementation of such haptic feedback technologies may provide interventionalists with important cues in steering tools accurately to target locations.
Advanced image guidance, sensing, and automation may also lead to improved targeting accuracy in needle-guided interventions. Wei et al. utilized 4D CT images to compensate for respiratory motion and plan an accurate path in an in vitro model with simulated respiratory motion. In a similar vein, Lei et al. registered intraoperative ultrasound to preoperative 3D CT and ultrasound, and demonstrated accurate puncture accuracy in an in vitro respiratory motion platform. Further, deep learning algorithms have enabled accurate real time needle tracking using ultrasound. , Beyond real-time imaging, many researchers have integrated embedded sensing such as optical fibers and electromagnetic probes into their needles for better tracking, shape reconstruction, and force sensing. In terms of automation, Kuntz et al. used electromagnetic tracking coils and registered them to preoperative CT scans to enable autonomous needle steering for lung biopsy in vivo in a porcine model. They also demonstrated significantly lower targeting errors than a manual bronchoscopy technique in ex vivo porcine lungs. These research efforts show promise for advancing the next generation of remote-controlled percutaneous interventions.
Remote-controlled endovascular robotics
Coronary and peripheral vascular intervention
Robotic systems have the potential to significantly improve endovascular interventions, particularly in coronary and peripheral vascular procedures, where precise navigation of catheters and guidewires is critical ( Table 2 ). Robotic assistance may enhance operator control and precision while reducing radiation exposure and physician physical strain.
One of the most prominent systems in this field was the CorPath series (Corindus Vascular Robotics, Waltham, Massachusetts), a remote-controlled endovascular robotic system ( Fig. 3 ). The use of CorPath 200 and its successor, the CorPath GRX system, has been well-documented in the context of both percutaneous coronary intervention (PCI) and peripheral artery disease (PAD) angioplasty.
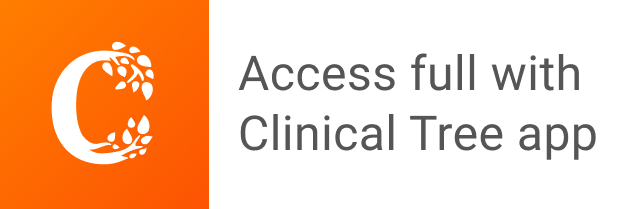