Abstract
Objective
This study aims to evaluate the viability of a hypothesis for selective targeting of skin cancer cells by exploiting the spectral gap with healthy cells using analytical and numerical simulation.
Methods
The spectral gap was first identified using a viscoelastic dynamic model, with the physical and mechanical properties of healthy and cancerous skin cells deduced from previous experimental studies conducted on cell lines. The outcome of the analytical simulation was verified numerically using modal and harmonic analysis. Finally, transient analyses were conducted analytically and numerically to evaluate the difference in vibrational response of healthy and cancerous cells when their resonant frequencies were closely matched. For analysis, we used healthy nucleus diameters of 3 µm, 5 µm and 7 µm, whereas 34 kPa was taken as the stiffness of healthy skin epithelial cells. Based on established trends, the nucleus-to-cytoplasm ratio was utilised to predict physical and mechanical properties as cells undergo neoplastic transformation.
Results
Analytical and numerical simulation revealed an approximate frequency difference of 50–100 KHz for the different nucleus diameters. The transient simulation revealed a significant difference in the growth rate of cancer cells’ vibration amplitude, which was 10 times greater than that of healthy cells.
Conclusions
This study highlights that cancer cells are more prone to resonance with tuned ultrasound frequencies, emphasising the need for detailed dynamic models incorporating the basement membrane’s influence and experimental validation.
Introduction
Many diseases in the human body are characterised by the uncontrolled growth and spread of underdeveloped cells, a condition where pre-mature new cells are formed and invade nearby tissue, thus disrupting normal bodily functions. Such a condition is commonly termed cancer. Approximately 14 million new cancer cases are reported each year. Human skin is an organ of the human body that is most exposed to the environment, which makes it prone to deterioration and diseases that evolve with time [ ]. Many nations have reported a rapid increase in melanoma and non-melanoma skin cancers, attributing this increase to excessive ultraviolet radiation exposure [ ]. Melanoma and non-melanoma skin cancers are particularly common in the southern hemisphere. Non-melanoma skin cancer (NMSC) increased by 22% in Australia alone between 2010 and 2015 [ ]. Brougham et al. [ ] reported an annual increased NMSC occurrence of 4.1% in New Zealand, in which basal cell carcinoma (BCC) contributed the most significant number of cases. Besides the threat to patient health and appearance, it also comes with a significant financial burden—approximately 90,000 non-malignant skin cancers are detected annually, costing approximately NZD 129.4 million. This cost is expected to rise to NZD 295 million by 2025 [ ].
The most prevalent type of skin cancer is BCC, which originates at the bottom of the epidermal layer. BCCs have a prevalence that is at least twice as high as that of squamous cell carcinomas, which are the second most frequently occurring form of skin cancer [ , ]. Basal cells generate new skin cells while pushing old cells to the skin surface for natural death. However, excessive UV radiation mutates the basal cells, producing pre-mature new cells and accumulating old cells on the skin’s surface. This aberrant cell accumulation leads to the development of cancerous tumours, as shown in Figure 1 .
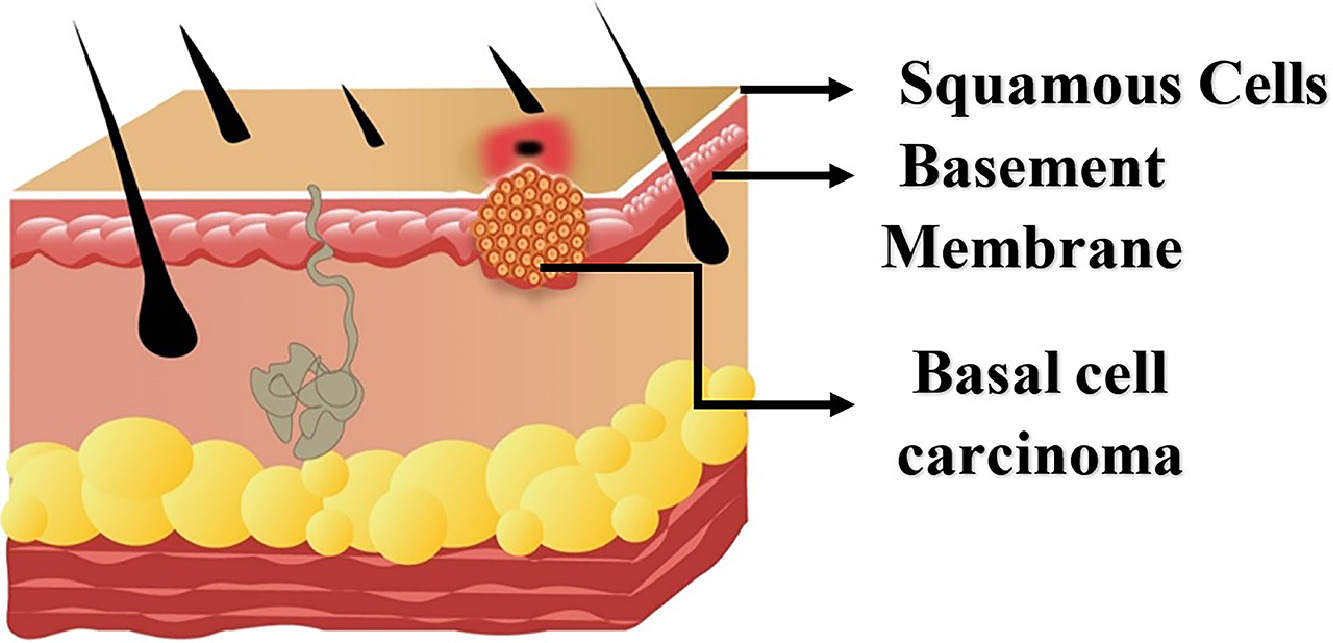
Numerous therapeutic techniques have been employed to treat cancers, including surgical excision, gamma radiation [ ], chemotherapy [ ] and cryotherapy. Among these, surgery and chemotherapy are invasive. Besides other hazards, tomophobia ( i.e. , fear of surgery) is the first instigator that has led to the investigation and development of non-invasive therapeutic techniques. However, chemotherapy comes with additional and inevitable complexities such as nausea, anaemia, bruising and hair loss [ ].
One of the first non-invasive therapeutic techniques developed soon after Roentgen’s discovery of X-rays in 1895 was the use of gamma radiation. Precisely controlled doses of radiation damage the DNA in cells; however, during treatment it can affect the DNA of healthy cells too, which becomes a breeding ground for secondary cancer [ ]. Another minimally invasive therapy currently used is cryosurgery or cryotherapy, which is rapid, cost effective and utilises sub-zero temperatures to freeze skin tumours. However, if not performed carefully it can cause local reactions such as blistering, permanent hypopigmentation, hypertrophic scarring, severe pain and tissue distortion [ , ].
In recent years, focused ultrasound therapy has rapidly gained the attention of researchers and clinical practitioners as a safe, non-invasive therapeutic technique. Various modalities of focused ultrasound are being investigated and some of them have reached clinical trials, such as high-intensity focused ultrasound [ ], ultrasound shockwave [ ] and contrast agent-based ultrasound drug delivery [ ]. Thermal ablation and inertial cavitation are two phenomena that occur upon application of focused ultrasound. When it is used with high intensity ( i.e. , I >100 w/cm 2 ), it becomes a challenge due to the accumulation of energy, particularly in sensitive areas such as the skin epidermis, which carries sensory receptors and experiences maximum pain.
The unwavering dedication and eagerness to reduce the suffering of cancer patients have led scientists to novel ideas for the treatment of cancer. In 2002, Johns [ ] coined the term ‘frequency response hypothesis’. He suggested that the sub-cellular structure can be resonated, causing structural changes. In 2008, Meir et al. [ ], while studying the alteration and function of cellular structures subjected to low-intensity therapeutic ultrasound, found that the intra-cellular displacement of various organelles in cells is significant and dominated by elastic behaviour, especially if the cytoplasm has lower viscosity. This relative displacement has been assumed to be due to differences in inertial response. In 2015, Fraldi et al. [ ] carried forward Meir et al.’s idea and presented a frequency-dependent hypothesis to target cancer selectively. They used the Voigt and Maxwell viscoelastic model to determine the spectral gap between healthy and cancer cells using experimentally verified data for the mechanical properties of cellular structure. The viscous response was modelled using virtual friction whereas inertial response was modelled in the form of added mass and simulated the response of various cells such as breast, kidney, thyroid, ovarian, bladder, lungs and prostate to determine the peak frequencies of cancerous cells and their healthy counterparts. A study with similar steps was performed by Heyden and Ortiz [ ] in 2016 at the California Institute of Technology (CA, USA) to target cancer cells, which they named ‘oncotripsy’. The main aim of this concept was to resonate cancer cells selectively by carefully tuning the excitation frequency to equal the natural frequency by exploiting the spectral gap between healthy and cancerous cells. This concept also aimed to overcome the reliance of therapeutic techniques on unique cancer biomarkers or the need for precise targeting [ ]. In this study by Heyden and Ortiz [ ], they modelled cellular constituents to be hyper-elastic, the difference in response to ultrasonic excitation at the natural frequency of healthy and cancerous liver cells (hepatocellular carcinoma); a gap of 36.6 KHz was revealed between the natural frequency of healthy and cancerous cells.
The same group published another article in 2017 [ ] investigating the influence of viscoelastic behaviour on the viability of oncotripsy; a slight reduction in natural frequency and an increase in the time of lysis was observed due to the inclusion of viscoelastic behaviour of cellular constituents, which further reinforced the viability of this technique. This concept was further investigated in the same lab by Mittelstein et al. [ ] in 2019; he used hepatocellular carcinoma as a subject and determined its natural frequency and response to various ultrasonic pulses.
Cancer is highly heterogeneous even within the same tumour. Hence, it is impossible to find a single set of parameters to target 100% of the cancer cells selectively; however, it can kill some while simultaneously stimulating the body’s natural defence mechanism to flood the tumour region with white blood cells to mop up the remaining tumour. However, all studies that aimed to selectively target cancer cells using ultrasound found that the resonant frequency lay in the tens to a few hundred KHz range. A comparison of differences in the resonant frequencies of healthy and cancerous cells of different types of cells that have been previously studied are summarised in Table 1 .
Ref. | Organ | Cell line | Resonant frequency |
---|---|---|---|
[ ] | Bladder cells | T24 (C) | 100 KHz |
HCV29 (H) | 221 KHz | ||
[ ] | Kidney cells | RC124 (C) | 166 KHz |
ACHN | 366 KHz | ||
[ ] | Breast cells | MCF7 (C) | 49 KHz |
A184A1 | 192 KHz | ||
[ ] | Breast cells | MDA-231 | 43 KHz |
MCF-10A | 139 KHz | ||
[ ] | Breast cells | MCF7 (C) | 36 KHz |
MCF10 | 140 KHz | ||
[ ] | Thyroid cells | S277 (C) | 103 KHz |
S748 (H) | 249 KHz | ||
[ ] | Prostrate cells | PC3 (C) | 131 KHz |
PZHPV7 | 221 KHz | ||
[ ] | Liver cells | Cancerous | 79 KHz |
Healthy | 43 KHz |
Recent independent studies have revealed that cancer cell stiffness varies in healthy cells at different stages of neoplastic transformation regardless of the measurement technique used. While Fred et al. [ ] concluded that cancer cells are consistently softer than their healthy counterparts, a literature review suggested that non-metastatic cancer stages show softer characteristics than healthy cells. However, once cancer cells progress to the metastatic stage, their stiffness increases, sometimes surpassing healthy epithelial cells, particularly in cases such as skin; this occurs due to increases in the stiffness of the surrounding extracellular matrix. This disparity presents an opportunity to explore the potential of targeting cancer cells based on their mechanical properties. Researchers have collated the stiffness values of various epithelial cell lines obtained through experimental methods in recent years, as outlined in Table 2 .
Investigator | Organ | Cell line | Stiffness (kPa) | |||
---|---|---|---|---|---|---|
Healthy | Immortal | Tumorigenic | Metastatic | |||
[ ] | Oesophagus | EPC2 | 4.7 | |||
CP-A | 3.1 | |||||
CP-D | 2.6 | |||||
– | – | |||||
[ ] | Breast | HMEC | 1.130 ± 0.060 | |||
hTERT | 0.720 ± 0.030 | |||||
HMLER | 0.410 ± 0.030 | |||||
MDA-MB231 | 0.660 ± 0.030 | |||||
[ ] | Breast | MCF10A | 13.69 ± 1.9 | |||
MCF-7 | 9.24 ± 1.39 | |||||
T47D | 8.39 ± 1.24 | |||||
MDA-MB-231 | 9.57 ± 1.38 | |||||
[ ] | Cervix | Ect1/E6E7 | 48.77 ± 3.33 | |||
HeLa | 25.25 ± 1.89 | |||||
SiHa | 21.09 ± 2.42 | |||||
CasKi | 26.73 ± 3.23 | |||||
[ ] | Lung | WI-38 | 47.52 ± 2.50 | |||
A549 | 15.50 ± 1.74 | |||||
H460 | 33.54 ± 1.10 | |||||
H1299 | 39.04 ± 4.45 | |||||
[ ] | Skin | – | ||||
WM35 | 3.44 | |||||
WM115 | 2.16 | |||||
WM793 | 2.29 | |||||
A375 | 0.85 | |||||
1205Lu | 1.10 | |||||
WM266-4 | 1.48 | |||||
[ ] | Breast | 184A1 | 2.24 | |||
MCF-7 | 1.21 | |||||
T47D | 1.02 | |||||
– | ||||||
[ ] | Bladder | HCV29 | 3.08 | |||
Hu609 | 3.14 | |||||
BC-372629 | 0.17 | |||||
T-24 | 0.77 | |||||
Hu456 | 0.83 | |||||
[ ] | Skin | HaCat | 34±3 | |||
UM-SCC47 | 25±2 | |||||
CAL33 | 6.2±0.6 | |||||
A375 | 1.6±0.2 |
The above literature reveals promising results and attests to the likelihood of selective targeting of cancer cells by exploiting their different mechanical and physical properties. However, skin cancer cells, being one of the most common and rapidly growing types of cancer, especially in the southern hemisphere, are yet to be studied. Like other cells, the magnitude of skin cells plays a crucial role in the dynamic nature of the cellular framework. It has been previously noted that alterations in nuclei size during neoplastic progression serve as a vital marker of malignancy. Numerous research endeavours have examined variations in the size and rigidity of epithelial cells as malignancy progresses—research conducted by Fuhrmann et al. [ ] discovered that the height of oesophageal epithelial cells ranges from 7 to 13 µm when transitioning from healthy to cancerous states. These data illustrate the average dimensions of epithelial cells in different organs, as investigated by various researchers.
This study aims to determine the spectral gap between healthy and cancerous skin cells using MATLAB for analysis and results verification using Ansys® Mechanical, Release 2024R2 for Finite element analysis. It also explores differences in the transient response of healthy and cancerous cells at different stages of neoplastic transformation when they are targeted at different excitation frequencies, thus contributing to efforts to reach effective cancer treatments.
Materials and methods
Data sets
Several studies have established that the nucleus exhibits a stiffness of around 10-times more and a density 30% higher than the cytoplasm [ , ]. However, enlargement of the nucleus due to increased chromatin is a biomarker for neoplastic transformation in cells. This has been incorporated into mathematical models using the nucleus-to-cytoplasmic (n:c) ratio, which compares the cross-sectional area of the nucleus and the cell and has been widely utilised in studies related to cancer [ , , ]. The n:c ratio is a critical factor that influences the response of the nucleus to the induced vibration, thus affecting the vibration of the nucleus within the cytoplasm. Analysis commenced with a standard n:c = 0.1 [ ] for a healthy cell, indicating that the nucleus occupies 10% of the cell’s volume. Any increase in the n:c ratio indicates an increase in the size of the nucleus due to cancer progression; however, the overall stiffness of the cellular structure reduces due to cancer progression, as established by various studies summarised in Table 2 . An increase in the n:c ratio has been assumed to cause an equivalent reduction in stiffness, i.e. , n:c = 0.2 corresponds to a 20% reduction in cytoplasm stiffness, consistent with the established morphological changes during neoplastic transformation. This analogy, as shown in eqns (1) and ( 2 ), facilitate the customisation of simulation parameters by considering simultaneous changes in the physical and mechanical properties of the cell as it transforms from a healthy to a cancerous state.
The average diameter of a nucleus in healthy cells typically falls within 5–9 µm. However, this diameter can increase significantly during neoplastic transformation, reaching up to 50 µm [ ]. Figure 2 shows the change in the size of cells with cancer as investigated by various researchers. For an ideal healthy cell, n:c = 0.1 [ ]; however, a study by Sebastian et al. [ ] found that the n:c ratio can increase up to 0.5 for non-melanoma cancer and up to 0.7 for melanoma cancer cells. A graphical explanation of n:c ratio ranging from 0.1 to 0.9 is shown in Figure 3 . In 2011 Samitha et al. [ ] studied the n:c ratio of epithelial skin cancer, where they found that it can reach up to 0.9 in cancer types like squamous cell carcinoma. Furthermore, they also observed that reductions in the size of the cytoplasm is also an early indication of malignancy. While studying increases in the size of skin cancer cells, Weder et al. [ ] found that the metastatic skin cancer cell size was approximately 50 µm. In contrast, normal human epithelial cells were almost half this size. This increase in size is primarily due to increased chromatin production, which increases the size of the nucleus.
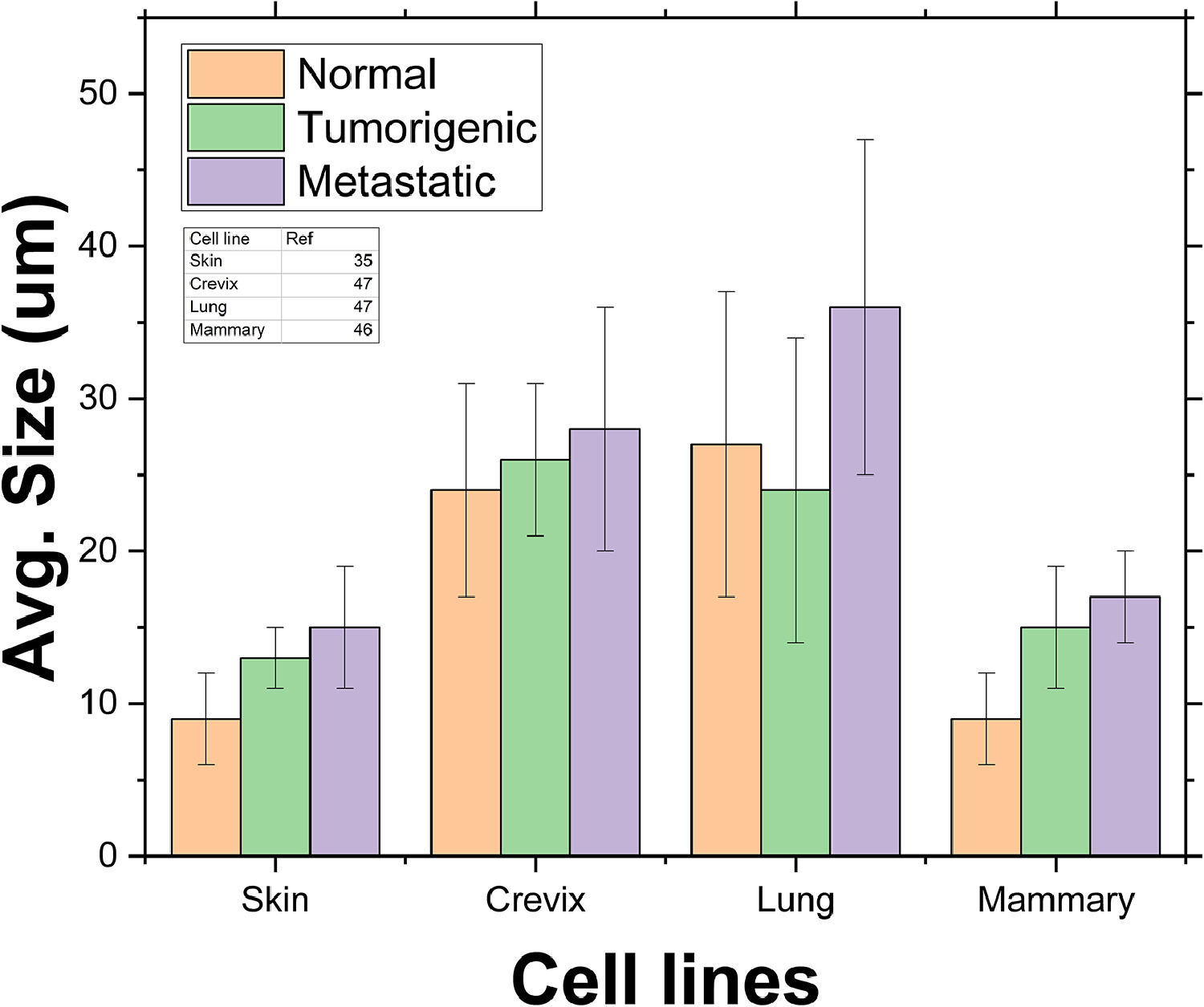

The analogy introduced by Heyden et al. [ ] for scaling sub-cellular structure properties in relation to the cancerous potential assumed that the properties of the cancerous cells are known. However, we employed the inverse of this analogy as we summarised the properties of healthy and cancerous skin epithelial cells reported by various researchers in their experimental studies, as shown in Table 2 .
The correlations between properties of healthy and cancerous cells are given below in eqns (1) and ( 2 ):
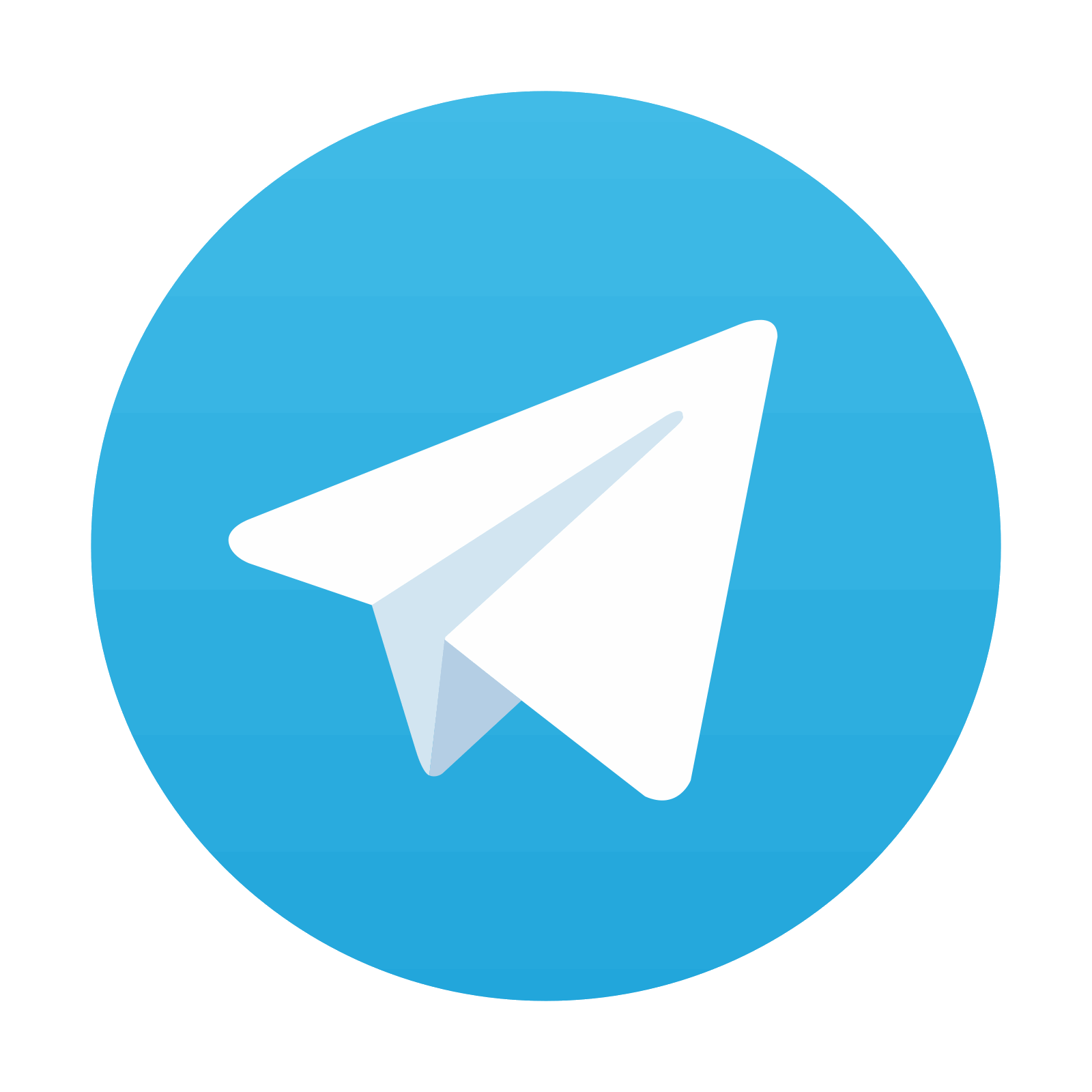
Stay updated, free articles. Join our Telegram channel
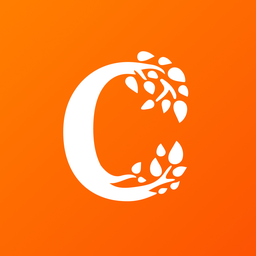
Full access? Get Clinical Tree
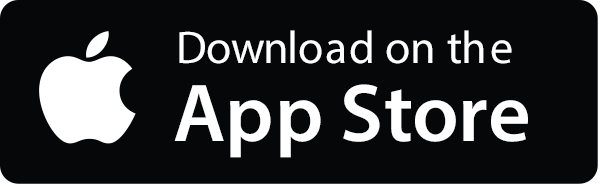
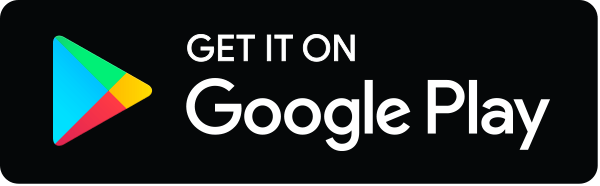
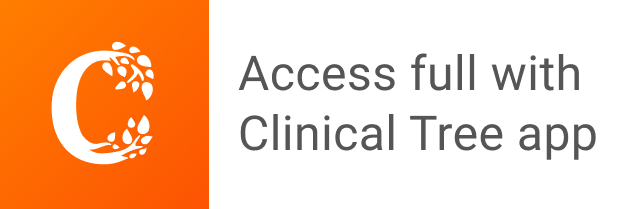