Abstract
Objective
The aim of this study was to investigate the mechanical effects of carotid shear wave elastography (SWE) in vivo as its effects on the arterial wall have not been thoroughly examined.
Methods
We evaluated the mechanical effects of carotid SWE in vivo in terms of the radial strain and strain rate to which acoustic radiation force impulses (ARFIs) expose the arterial wall, and compared them with the strain and strain rate induced by arterial pulsation in 13 healthy study subjects (seven individuals 20–35 y of age and six individuals 50–65 y of age). Additionally, we explored whether mechanical effects vary with timing of ARFI and subject age.
Results
The young cohort was found to have, compared with the old cohort, a higher diastolic ARFI-induced peak strain ( p = 0.002) and peak strain rate ( p = 0.001), and a lower diastolic ARFI-induced peak negative strain rate ( p = 0.013). When comparing cardiac phases, diastolic ARFIs were found to induce a lower peak negative strain rate than systolic ARFIs ( p = 0.006). Importantly, ARFI-induced peak strain was lower than that caused by arterial pulsation in both age cohorts ( p < 0.0001). The ARFI-induced peak strain rate was slightly higher than that caused by arterial pulsation at rest but lower than published exercise data. The ARFI-induced peak negative strain rate was similar to that caused by arterial pulsation.
Conclusion
Our results indicate that arterial SWE does not expose the arterial wall to any higher strain or strain rate than is experienced during normal arterial pulsation. Further research is required to validate the results in arteries containing vulnerable plaques.
Introduction
Shear wave elastography (SWE) is a non-invasive ultrasound technique for mapping tissue stiffness. SWE has been applied clinically to improve the characterization of breast lesions [ , ], liver fibrosis [ ] and thyroid nodules [ ], and has exhibited promising results in the assessment of several pathological conditions of various soft tissues, including the prostate [ ], lymph nodes [ ], tendons [ ], skeletal muscle [ ], nerves [ ], ligaments [ ], cornea [ ] and myocardium [ ]. SWE quantifies tissue stiffness by means of an acoustic radiation force impulse (ARFI), which generates a shear wave that propagates through the tissue. The shear wave is subsequently tracked using plane-wave or ultrafast imaging [ ], where a higher velocity indicates stiffer tissue. The shear modulus, μ , of the tissue can be derived from the local shear wave propagation velocity. Large isotropic organs where the smallest dimension significantly exceeds the shear wavelength (typically in the order of millimeters) can be approximated as having infinite extent. In such cases, the group velocity of the shear wave, c g , can be used to estimate the shear modulus, μ , using the relationship <SPAN role=presentation tabIndex=0 id=MathJax-Element-1-Frame class=MathJax style="POSITION: relative" data-mathml='μ=ρcg2′>𝜇=𝜌𝑐2𝑔μ=ρcg2
μ = ρ c g 2
. However, this assumption is not valid for smaller structures such as arteries due to geometric wave velocity dispersion. In these cases, frequency-dependent phase velocity must be analyzed to accurately determine the shear modulus of the tissue. Phase velocity analysis based on the propagation of guided waves in a plate has been used in previous studies to quantify arterial stiffness [ ]. In this approach, the geometry of the artery also influences the dispersion curve and thus affects the estimated modulus [ , ].
Given the association between changes in the elastic properties of the arterial wall and atherosclerotic lesions with major clinical challenges such as stroke, heart failure and hypertension [ ], cardiovascular applications have become an area of growing interest for SWE techniques. In particular, the incremental diagnostic value of carotid artery SWE has been recently investigated in obstructive coronary artery disease [ ], spontaneous coronary artery dissection [ ], type 2 diabetes [ ], alcoholic fatty liver disease [ ] and atherosclerotic plaque characterization [ , ]. The utility of SWE in the characterization of atherosclerotic plaque derives from the detectable difference in stiffness between calcified regions and soft or hemorrhagic tissue [ , ].
As ARFI generation necessitates higher peak pressure and longer pulse duration than conventional B-mode imaging, the application of SWE in the arterial wall and near vulnerable plaque may raise safety concerns. Finite in silico element model simulations of ARFIs in plaques suggest that the ARFI-induced strain is lower than the strain associated with blood pressure [ ]. However, SWE tissue compression occurs in short time frames compared with arterial pulsation, resulting in higher rates of strain. Both arterial wall strain and the rate of strain are crucial components of cellular mechanotransduction and re-modeling [ , ]. Although ARFI sequences must adhere to regulatory limits on thermal indices, mechanical indices and average intensities, the potential effects of mechanical stress in the arterial wall remain relatively unexplored.
Ex vivo studies of the porcine aorta have found arterial SWE to induce strain an order of magnitude lower than that induced by arterial pulsation [ , ]. However, assessing the mechanical effects ex vivo entails multiple limitations, such as no surrounding tissue, un-pressurized vessels, and no blood pressure dynamics throughout the cardiac cycle. Additionally, porcine aortas differ significantly from human carotid arteries, with a thicker intima-media [ ], larger lumen diameters [ ] and a lower elastic modulus [ ]. These vessels are also anatomically distinct due to their unique hemodynamic roles [ ]. The aforementioned limitations in previous SWE safety studies motivate further investigation of ARFI-induced strain and strain rate patterns in the human carotid artery that, to the authors’ knowledge, remain unexplored.
In this study, we compared ARFI-induced arterial strain and strain rate with the inherent strain and strain rate induced by arterial pulsation. Strain and strain rate by arterial pulsation are commonly used to assess the dynamic behavior of arterial walls to provide insight into the mechanical properties of arteries [ ]. In ultrasound imaging, arterial strain and strain rate, typically estimated by block-matching techniques such as speckle tracking, have been shown to differentiate asymptomatic from symptomatic plaques [ ]. However, the advantage of SWE over estimation of strain by arterial pulsation as a diagnostic tool is the potential to provide a more direct and quantitative measurement of arterial stiffness by tracking induced wave velocities that are directly related to mechanical properties. Arterial strain is a more indirect measure of arterial stiffness, primarily determined by the pulsatile motion of the arterial walls and influenced by hemodynamic factors such as blood pressure, heart rate and arterial geometry. In addition, SWE may allow a more localized assessment of stiffness compared with strain by arterial pulsation, which may be of particular importance for characterizing plaques in a localized region.
The aim of this study was to investigate the mechanical effects of carotid SWE in vivo in terms of ARFI-induced strain and strain rate, and to compare them with the strain and strain rate induced by arterial pulsation. Furthermore, this study explored whether mechanical effects vary with ARFI timing in relation to the cardiac cycle and study subject age, as two approaches to study the dependence of the mechanical effects on differences in arterial stiffness.
Methods
A total of 13 volunteers from two age groups (20–35 and 50–65 y of age) were recruited locally ( Table 1 ). Written informed consent was obtained from each participant. The study was approved by the local ethics committee (Swedish Ethical Review Authority, diary no. 2021-06991-01). Study subjects were excluded if they had previously undergone or were currently undergoing treatment for cardiovascular disease. A 12-lead ECG was recorded for 10 s using QRS-Card Cardiology Suite 4.06 (Pulse Biomedical Inc, King of Prussia, PA, USA) and assessed by an experienced cardiologist to ensure the absence of exclusion criteria.
Characteristic | Young | Old |
---|---|---|
Age (y) | 28 ± 3 | 58 ± 4 |
Sex | 3 female, 4 male | 2 female, 4 male |
Weight | 74 ± 16 kg | 85 ± 15 kg |
Height | 175 ± 10 cm | 179 ± 12 cm |
Resting heart rate | 69 ± 9 bpm | 70 ± 11 bpm |
Systolic blood pressure | 134 ± 18 mmHg | 136 ± 21 mmHg |
Diastolic blood pressure | 83 ± 12 mmHg | 83 ± 15 mmHg |
Two sequential ultrasonic examinations were performed on each volunteer in the supine position. First, B-mode ultrasound speckle tracking was employed to quantify the radial strain and strain rate caused by arterial pulsation, measured perpendicular to the long axis of the artery and axially with respect to the transducer. Second, ARFIs were performed in the anterior wall of the carotid artery and the radial strain and strain rate of the subsequent propagating waves were measured. It should be noted that we only reported radial strain induced by arterial pulsation and not strain in the longitudinal direction of the arterial wall to facilitate comparison with ARFI-induced strain, which occurs in the radial direction (axial direction with respect to the transducer). Figure 1 outlines ARFI and arterial pulsation data acquisition.
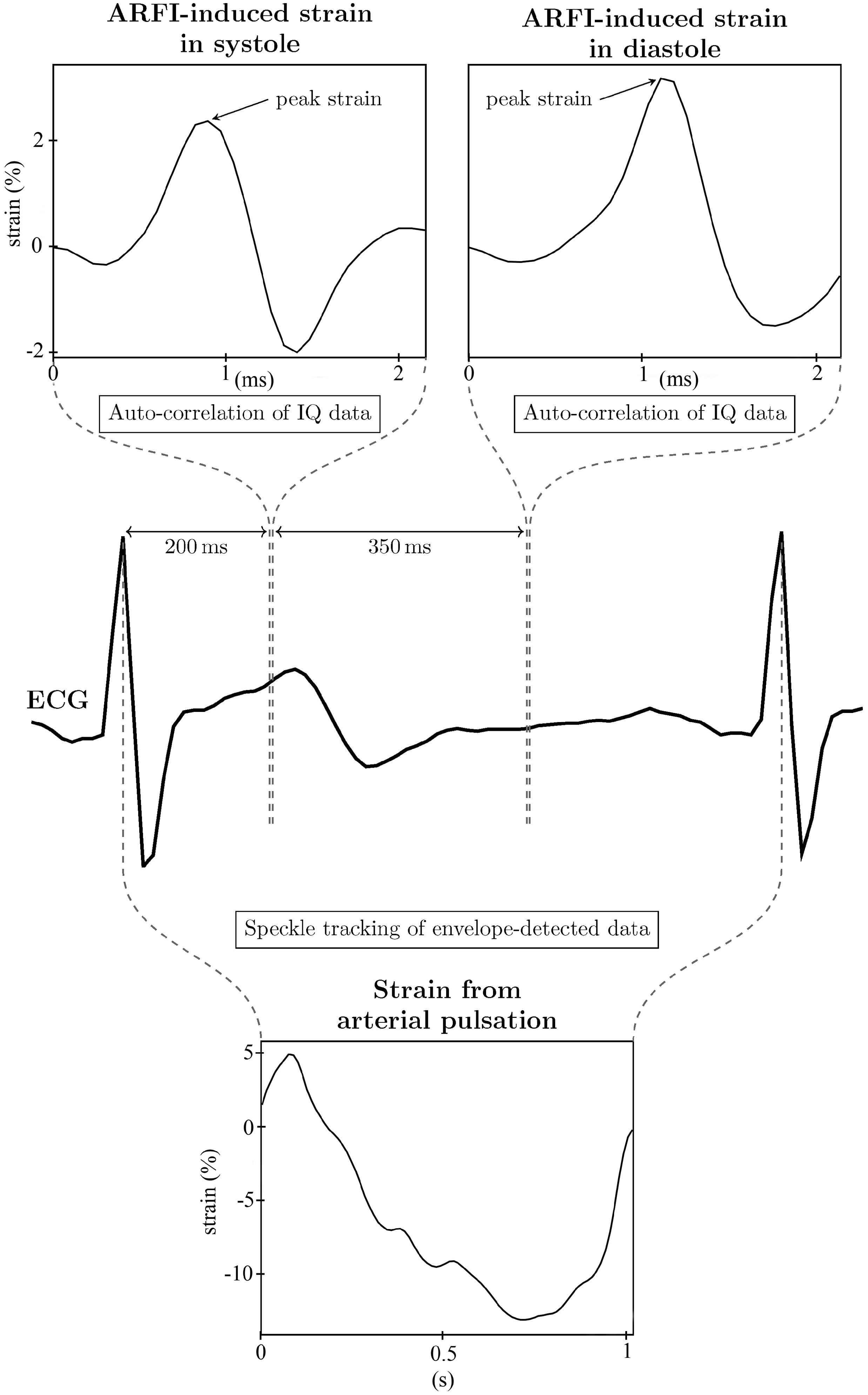
Estimation of strain and strain rate induced by arterial pulsation
A GE Vivid E9 ultrasound machine (GE Healthcare, Horten, Norway) equipped with a 9 L linear array transducer was used at 10 MHz center frequency to obtain B-mode ultrasound long-axis views of the left and right common carotid artery ( Fig. 2 a, 2 b). Images were recorded at 81 frames per second for three cardiac cycles without compounding. Imaging was performed by an experienced carotid ultrasound operator.
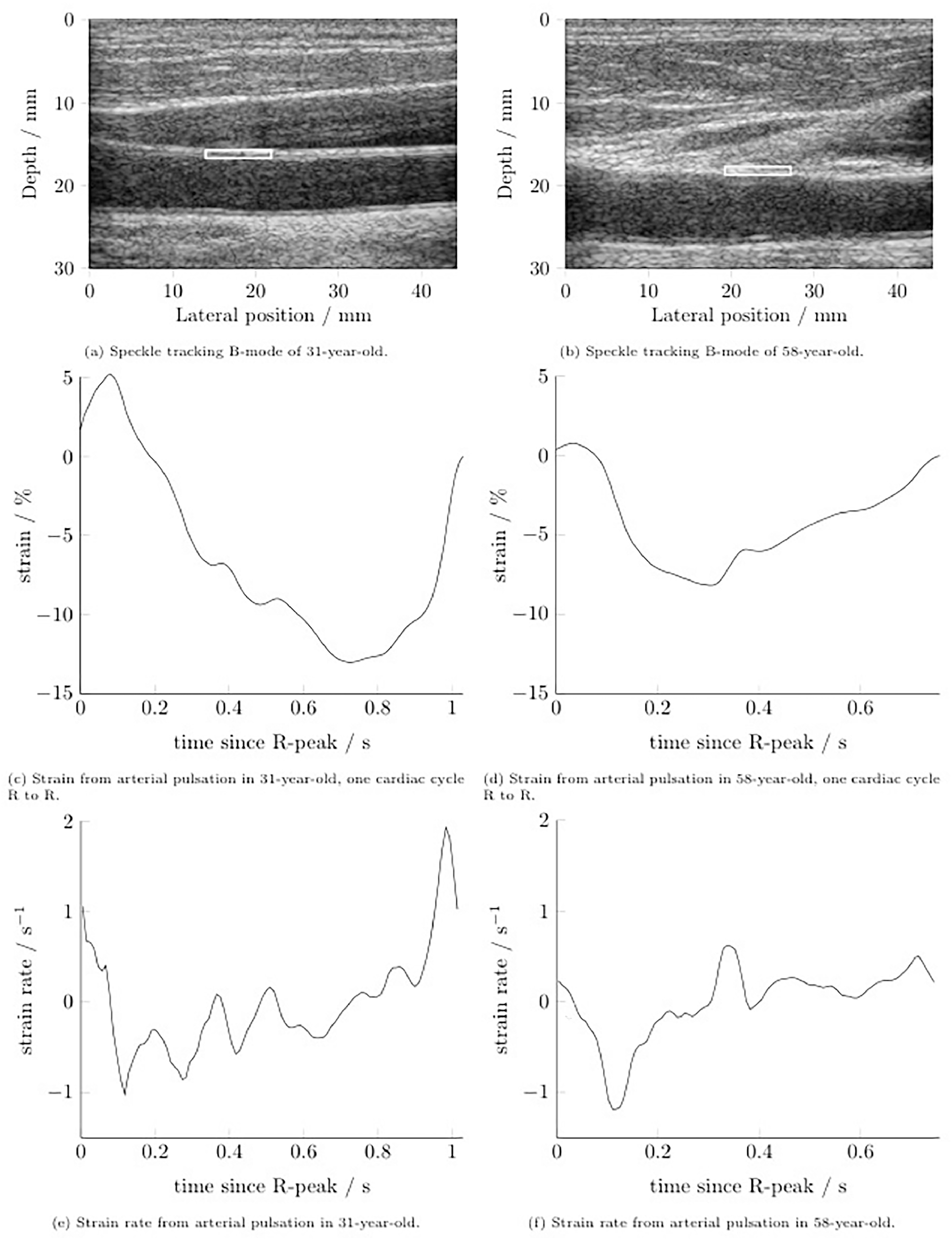
An ultrasound speckle-tracking algorithm previously tuned and validated for the arterial setting [ ] was used to assess the carotid radial strain and strain rate induced by arterial pulsation. Speckle tracking was performed on the envelope-detected data using a kernel size of 0.7 × 0.2 mm (lateral by axial with respect to the transducer). Radial strain was obtained through spatial linear regression after averaging the cumulative displacement maps longitudinally in a region of interest (ROI) measuring 8.0 × 1.0 mm (lateral by axial with respect to the transducer), which was manually placed in the middle of the anterior wall ( Fig. 2 a, 2 b). Radial strain rate was assessed using the finite difference approximation of the time-dependent strain.
Estimation of ARFI-induced strain and strain rate
A Verasonics V1 system (Verasonics, Kirkland, WA, USA) was used to generate ARFIs and image the subsequent wave propagation for the estimation of ARFI-induced strain and strain rate. A linear array transducer (L7-4, Philips Healthcare, Andover, MA, USA) was connected to the Verasonics V1 system and positioned to view the long axis of the carotid artery ( Fig. 3 a, 3 b). A 12-lead ECG (Pulse Biomedical Inc) was used in conjunction with custom-built hardware to identify ECG R-peaks in order to trigger ARFIs.
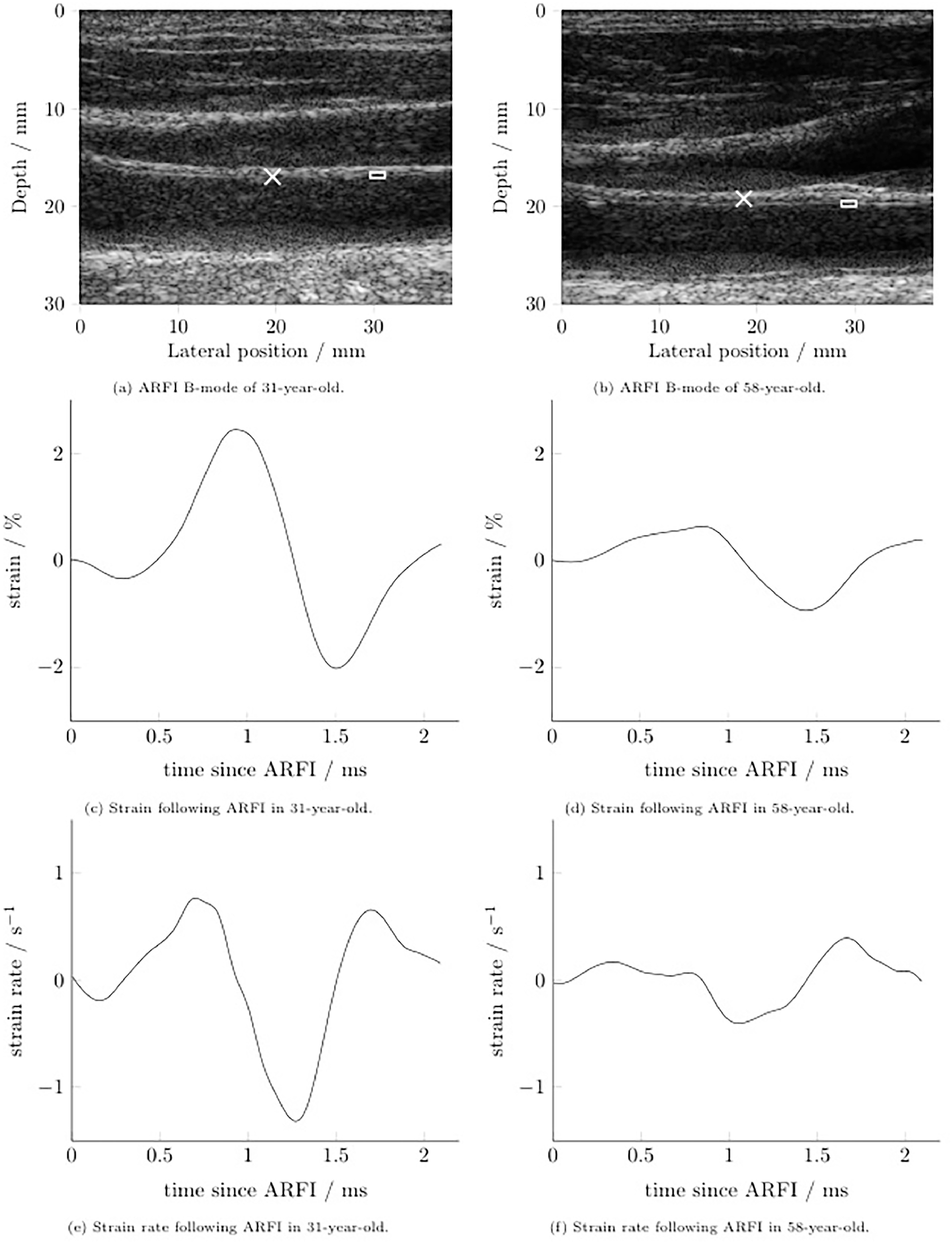
The Verasonics system was operated by the same experienced carotid ultrasound operator who performed B-mode free-hand scanning for speckle tracking analysis. In each common carotid artery of each study subject, three separate ARFI series were performed in the anterior wall, approximately 3 cm from the carotid bulb. Each ARFI series consisted of eight ARFIs: four triggered in systole and four triggered in diastole. Systolic ARFIs were performed 200 ms after an R-peak trigger and diastolic ARFI 550 ms after an R-peak trigger to coincide with arterial systolic and diastolic pressure times, respectively. In total, 2 × 3 × 8 = 48 ARFIs (left and right carotids, three ARFI series per carotid, eight ARFIs per series) were performed per study subject.
ARFIs were generated at 180 V peak-to-peak voltage and 5 MHz center frequency for 200 µs. Beam width was maintained at a constant f-number (ratio between focal depth and aperture width) of f/1.5 by adjusting the aperture width, i.e. , the number of transmitting piezoelectric elements, based on the depth of the carotid wall. Each beam was laterally centered on the transducer.
Wave propagation in the carotid wall was imaged at 6.4 MHz center frequency and 12.5 kHz pulse repetition frequency using plane-wave imaging. Fifty plane-wave images (4 ms) were collected per ARFI. Plane-wave imaging radio-frequency samples were stored and processed into in-phase/quadrature (IQ) data using Verasonics V1 built-in beamforming processes in Matlab R2012b (MathWorks, Natick, MA, USA). Remaining signal processing was performed in Matlab R2015a. ARFI-induced strain and strain rate of the arterial wall were assessed in a ROI measuring 1.5 mm (laterally) × 0.6 mm (axially with respect to the transducer), located 10 mm laterally from the ARFI focal point ( Fig. 2 a, 2 b). The signal-processing chain from IQ data to strain and strain rate has been described in a previous publication [ ], to which we refer for details. In brief, tissue displacement in the radial direction was estimated using Loupas et al.’s 2-D auto-correlation method [ ]. At each lateral pixel, a linear strain curve was least-squares fitted to the time-dependent cumulative radial displacement through the wall. Strain rate was estimated by differentiating strain through a finite difference method.
Statistical analysis
ARFI-induced peak strain was compared with peak strain from arterial pulsation using two-sample t -tests. This was done for both diastolic and systolic ARFIs in each age cohort and in the aggregate group. The same t -tests were performed for peak strain rate and peak negative strain rate, resulting in 2 × 3 × 3 = 18 (two cardiac phases, three measured parameters, two age cohorts and aggregate) tests. Two-sample t -tests were employed to compare the peak strain, peak strain rate and peak negative strain rate between the old and young cohorts. This was done for ARFI-induced strain in systole, ARFI-induced strain in diastole and strain from arterial pulsation, totaling 3 × 3 = 9 (three strain categories, three measured parameters) tests. The distributions were determined to be approximately normally distributed via comparison between mean and median, as well as visual assessment. Finally, induced strain from systolic ARFI was compared with induced strain from diastolic ARFI in the combined study subject group by means of three paired t -tests for peak strain, peak strain rate and peak negative strain rate. A total of 27 significance tests were carried out. Bonferroni correction was employed to address multiple testing, resulting in a significance level of 0.0019 for each test. The consistency of strain and strain rate within acquisitions from the same artery was estimated by calculating the coefficient of variation (CV), expressed as the percentage relative standard deviation (ratio of the standard deviation to the mean) of repeated measurements per artery (left and right carotid artery) in ARFI datasets in diastole and systole (four repeated pushes) and three repeated measurements by speckle tracking, and then averaged over the entire study population.
Results
Speckle tracking of the carotid artery during arterial pulsation is demonstrated in Figure 2 (a–f). Sample B-mode images of a young and an old study subject, along with the regions of interest, can be seen in Figure 2 (a, b). Figure 2 (c, d) show the calculated strain across the heart cycle for these two cases. Figure 2 (e–f) show the respective strain rates.
Similarly, strain and strain rate estimations of the ARFI-induced wave propagation in systole in the same two study subjects are demonstrated in Figure 3 (a–f). Sample B-mode images, ARFI focal points and regions of interest can be seen in Figure 3 (a, b). Figure 3 (c, d) shows the calculated strain in the ROI. Figure 3 (e–f) shows the respective strain rates.
Table 2 presents the ARFI-induced peak strain, grouped by cardiac phase (systole/diastole) and age cohort, along with the peak strain induced by arterial pulsation. The same measurements are presented for peak strain rate and peak negative strain rate. It could be seen that the ARFI-induced peak strain was lower than that caused by arterial pulsation in both cohorts, and that the ARFI-induced peak strain rate and peak negative strain rate are similar to that caused by arterial pulsation.
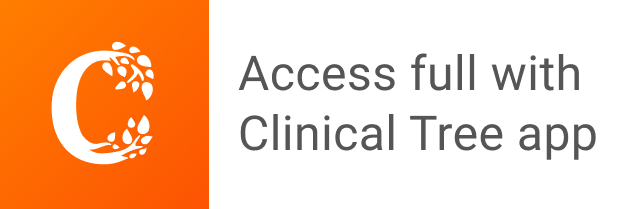