The process of aging in the brain is reflective of various factors including the environment, lifestyle, genetics, and management of concurrent chronic conditions. Aging in the brain leads to observable structural changes on neuroimaging, such as brain volume reduction, neuronal atrophy, and synaptic loss, which affect higher cognitive functions. Positron emission tomography imaging can help visualize these changes earlier before structural changes even take place and the associated decline in brain function, revealing important insights into how the brain ages and the impact on neural connectivity and cognitive abilities.
Key points
- •
Aging in the brain leads to observable structural changes on neuroimaging, such as brain volume reduction which affect higher cognitive functions. Positron emission tomography (PET) imaging can help visualize these changes earlier before structural changes even take place and the associated decline in brain function, revealing important insights into how the brain ages and the impact on neural connectivity and cognitive abilities.
- •
Structural magnetic resonance imaging (MR imaging) shows significant age-related reductions in gray matter volume, cortical thickness, and white matter volume, especially in cognitive regions like the frontal and temporal lobes. Advanced MR imaging techniques like diffusion tensor imaging and diffusion kurtosis imaging reveal microstructural deterioration, correlating with cognitive decline.
- •
Functional MR imaging studies show that normal aging leads to reduced connectivity within the default mode network and altered brain activation patterns, with older adults recruiting additional frontal regions to compensate for declines in other areas.
- •
Glucose metabolism decreases progressively with aging in various brain regions. Studies show a significant decline in whole brain CMR GLC in older adults, notably in the frontal, temporal, parietal, and occipital lobes, as well as the insula, anterior cingulate cortex, putamen, and thalamus, with lesser decrease in the cerebellum and brainstem.
- •
Tau and amyloid PET imaging detect protein accumulations linked to aging and neurodegenerative diseases. Tau-PET tracers show higher uptake in Alzheimer’s disease patients’ temporal and parietal lobes, while amyloid-PET tracers reveal increasing beta-amyloid plaques with age.
Introduction
The process of aging in the brain is reflective of various factors including the environment, lifestyle, genetics, and management of concurrent chronic conditions. While there is no clear consensus on a proper measure for brain aging, this process often coincides with cognitive decline, where there is a reduction in processing speed, executive functioning, and episodic memory. Structural changes commonly occur, such as a reduction of brain volume, sulcal widening, and expansion of the ventricular system. With a higher incidence of neurodegenerative disorders in older populations, many pathologic aging processes leverage cognitive tests and atrophy rates to distinguish healthy brain aging from neurodegenerative disease. , , , Woodworth and colleagues determined healthy brain aging based on neuropsychological tests; lower performance on these tests correlated with white matter (WM) lesions, which were found in older participants without dementia. Certain studies use the mini-mental status examination, as scoring system for healthy brain aging, a score below 24 indicates a lower hippocampal volume and neurodegenerative disease. A score of 24 and above is characteristic of healthy aging in older individuals. Some studies score based on the digit symbol substitution test (DSST) and modified mini-mental status examination (3MS). The average DSST score for healthy aged participants was 36.3. The 3MS is scored from 0 to 100, where higher scores indicate better cognition; a mean score of 91.6 on the 3MS to be considered healthy brain aging .
In addition to neuropsychological testing, neuroimaging modalities allow for the observation of in vivo brain changes associated with the normal aging process. Initially, computed tomography (CT) was the most widely used modality in distinguishing certain neurodegenerative disease features, such as WM lesions and infarcts, but was limited by poor soft tissue visualization. , Today magnetic resonance imaging (MR imaging) and positron emission tomography (PET) provide further information on detailed structural and physiologic changes, respectively. , This review aims to highlight the changes associated with nonpathologic brain aging and discuss the relative value of PET and MR imaging, in the aging process.
The aging brain
Aging in the brain is marked by numerous molecular and cellular changes influenced by genetics, neurotransmitters, and hormone fluctuations. Aging is associated with extensive alterations in DNA methylation, telomere shortening, histone loss, and global chromatin remodeling. RNA modification and the regulation of noncoding RNAs play crucial roles in posttranscriptional processes contributing to cellular senescence. Senescent cells, characterized by a proinflammatory state and a specific secretome of cytokines, chemokines, proteases, and growth factors, undergo permanent growth arrest, preventing further cell division. Oxidative stress, manifested as an increase in reactive oxygen species, exacerbates with age, leading to a decline in antioxidant defenses and resulting in DNA, protein, and lipid damage, thereby impairing cellular function.
These cellular changes manifest as observable structural changes in neuroimaging. Brain volume decreases with age, at a rate of about 5% per decade after age 40, and potentially accelerating after age 70. Neuronal atrophy, characterized by the shrinkage of neurons and loss of dendritic spines, affects both synaptic connections and neural connectivity. Synaptic loss hampers neuron-to-neuron communication, leading to cortical thinning and impacting higher cognitive functions. This process is accompanied by an increase in the activity of glial cells, such as astrocytes and microglia, resulting in inflammation and scarring. Demyelination contributes to WM shrinkage, slowing neural signal transmission. Synaptic plasticity and neural connectivity also become less efficient with age, with significant connectivity loss in regions such as the hippocampus and prefrontal cortex. Although the brain attempts to compensate by reorganizing existing networks, these compensatory mechanisms are often insufficient to fully restore function.
In addition to these neuron-level changes, the blood–brain barrier (BBB) alterations also occur with aging. The BBB plays a significant role in blocking unwanted toxins and pathogens from entering the brain. However, with age, this protective barrier deteriorates due to structural changes in endothelial cells, increased inflammation, and accumulated oxidative stress, which weakens the tight junctions. This reduction in integrity allows potentially harmful substances to enter the brain, leading to further damage and contributing to age-related changes and possible neurologic disorders.
Studies have demonstrated that crystallized abilities—accumulated skills and memories from past cognitive processing—improve until around age 60, after which they remain stable until about age 80. In contrast, fluid abilities, which involve the manipulation and transformation of information in the current environment, show a consistent decline from age 20 to age 80. The interplay between BBB deterioration and these cognitive changes underscores the complex nature of brain aging, where both protective mechanisms and cognitive functions are gradually compromised.
Magnetic resonance imaging
MR imaging is a widely used neuroimaging modality due to its nonionizing nature and enhanced image resolution. MR imaging’s multiplanar images with high spatial resolution shows improved distinction between various tissues, including WM, gray matter (GM), and the ventricular system. , , Various MR imaging sequences have been developed over decades, including structural imaging sequences like T1-weighted imaging (T1WI), T2-weighted imaging (T2WI)/fluid-attenuated inversion recovery (FLAIR), diffusion-weighted imaging (DWI), and susceptibility-weighted imaging (SWI). Functional MR imaging (fMR imaging) includes resting-state fMR imaging (rsfMR imaging) and task-related fMR imaging (tfMR imaging). Additional sequences include diffusion tensor imaging (DTI) and arterial spin labeling ( Table 1 ).
Imaging Sequence | Description and Usage | Key Findings/Changes with Age |
---|---|---|
T1-weighted imaging | Structural imaging assessing brain structures such as cortical thickness, GMV, WMV, ventricle size, and CSF spaces. | Most sensitive modality for assessing brain aging. Shows common age-related changes like atrophy and increased CSF spaces. Early growth from birth, peaks later in childhood, gradual decrease from late childhood onwards. Progressive thinning in adulthood, especially in prefrontal and temporal lobes. Noticeable changes in old age. |
T2-weighted imaging | Used for assessing WM lesions volumes and microstructural compartments. | Detects WMH, which is part of normal aging and certain pathologies. WMH increases with age, particularly in mid to late adulthood. |
Diffusion-weighted imaging | Quantitative method assessing WM microstructure through Brownian motion of water molecules. | Assesses structural connectivity. Decline in FA and increases in mean diffusivity noted with aging, impacting cognitive and motor functions. |
Susceptibility-weighted imaging | Used to assess for microbleeds and venous vessels. | Least predictive modality for brain aging. |
Diffusion tensor imaging | Constructs brain fiber tracts by mapping the direction and magnitude of water diffusion. | Employs tractography to map fiber tracts. Reductions in FA are modest in early adulthood, increase over time. Maximum effect noted in prefrontal areas. Early microstructural changes in anterior brain regions. |
Resting state functional MR imaging | Assesses connectivity between brain regions with spontaneous amplitudes. | Shows reduced connectivity within the DMN with aging. Altered DMN connectivity in older adults, especially notable in mid to late adulthood. |
Task-related functional MR imaging | Measures task-based brain activation patterns. | Compensatory mechanisms more pronounced in mid to late adulthood. Shifts in activation patterns during cognitive tasks observed in older adults. |
All these MR imaging modalities offer valuable insights into the aging brain, as leveraged by various studies. In the largest study to date, Xiong and colleagues compared several machine learning algorithms to predict brain age using T1WI, T2WI, DWI, SWI, rsfMR imaging, and tfMR imaging. In T1WI, they measured brain structures, while in T2WI, they measured WM lesion volumes. DWI was used to assess WM microstructural compartments and structural connectivity between different brain regions. SWI assessed microbleeds and venous vessels. fMR imaging was used to evaluate connectivity between brain regions, with rsfMR imaging focusing on spontaneous amplitudes and tfMR imaging on task-based amplitude responses.
Xiong and colleagues found that the most sensitive modality for assessing brain aging was T1WI, followed by DWI. In contrast, SWI was the least predictive. They suggested that the higher sensitivity of T1WI and DWI might be due to the broader selection of features and imaging-derived phenotypes compared with other modalities. Anatomic structures assessed by T1WI include cortical thickness, gray matter volumes (GMVs) (both cortical and subcortical), white matter volume (WMV), ventricle size, and cerebrospinal fluid (CSF) spaces, which show common aging-related changes due to atrophy. Overall, whole brain volume decreases with age, leading to increased CSF and ventricular spaces, most prominently in the lateral ventricles. ,
Gray Matter
Various methods have been used to assess GM in aging populations, with automated segmentations being the most common. These segmentations report measurements in cortical GMV and cortical thickness. Some studies suggest that cortical thickness is a more accurate measure of GM age-related changes as GMV depends on both surface area and cortical thickness, and surface area does not reliably predict normal brain aging due to sex differences. However, surface area measurement is gaining interest for studying brain regions in disease processes. ,
The trajectory of cortical thickness rapidly increases during infancy (1–6 years), especially in sensory and motor regions, then plateaus and declines through late childhood (6–12 years), starting in the prefrontal cortex. Adulthood is marked by progressive thinning, particularly in the prefrontal and temporal lobes, affecting cognitive and executive functions. In old age, cortical thickness continues to decline, significantly impacting cognitive and motor functions. , Age-related decline in cortical thickness is widespread but more pronounced in the frontal and parietal regions compared with the temporal and occipital regions.
Similarly, cortical GMV undergoes substantial early growth from birth, peaking later in childhood, and then gradually decreases from late childhood onwards. During adolescence, synaptic pruning reduces volume, particularly in the prefrontal cortex. In adulthood, a gradual decrease in cortical GMV is observed, especially in regions involved in higher cognitive functions like the frontal and temporal lobes. In old age, this reduction becomes pronounced, correlating with cognitive decline and an increased risk of neurodegenerative diseases ( Figs. 1 and 2 ). ,
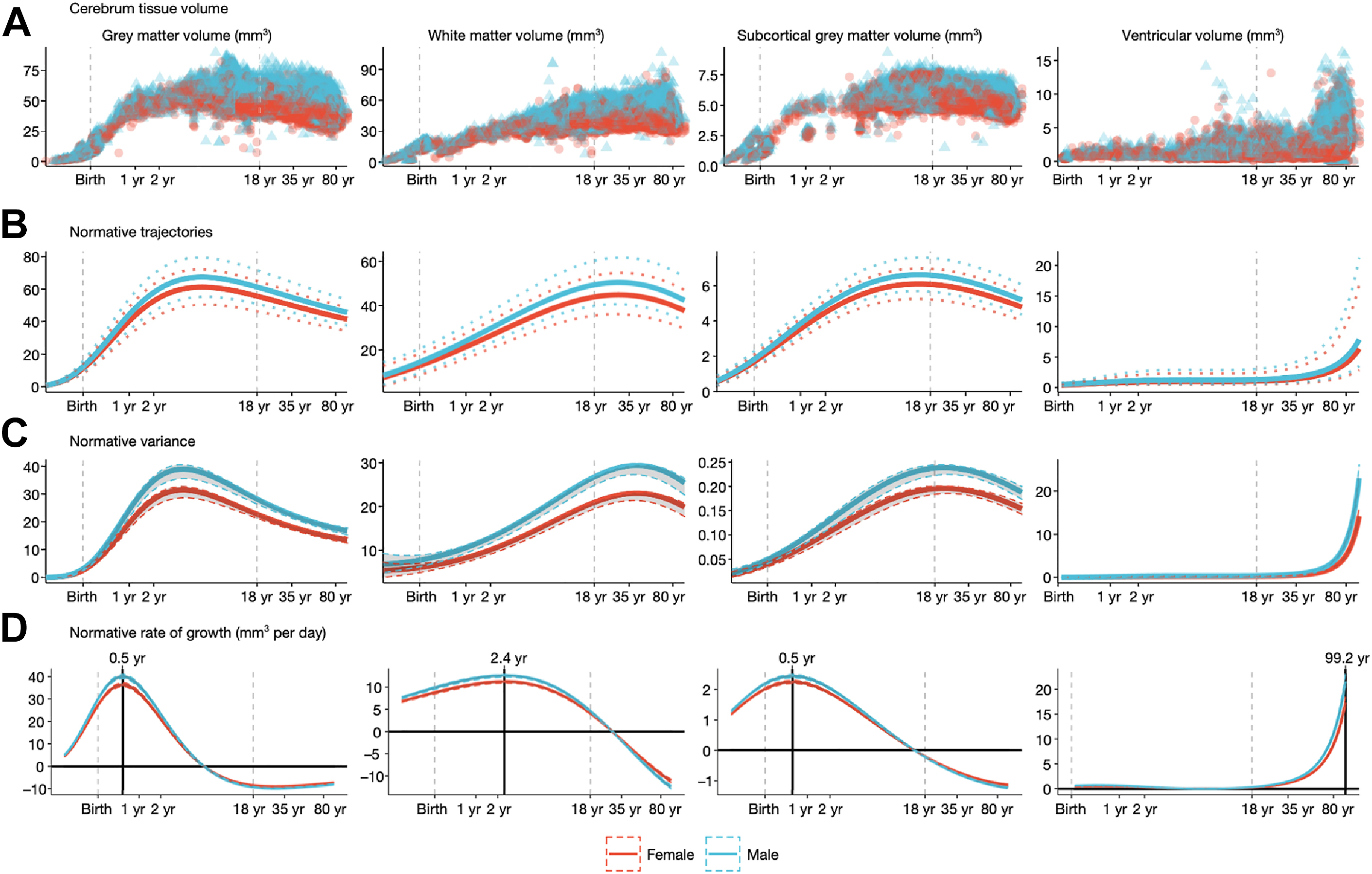
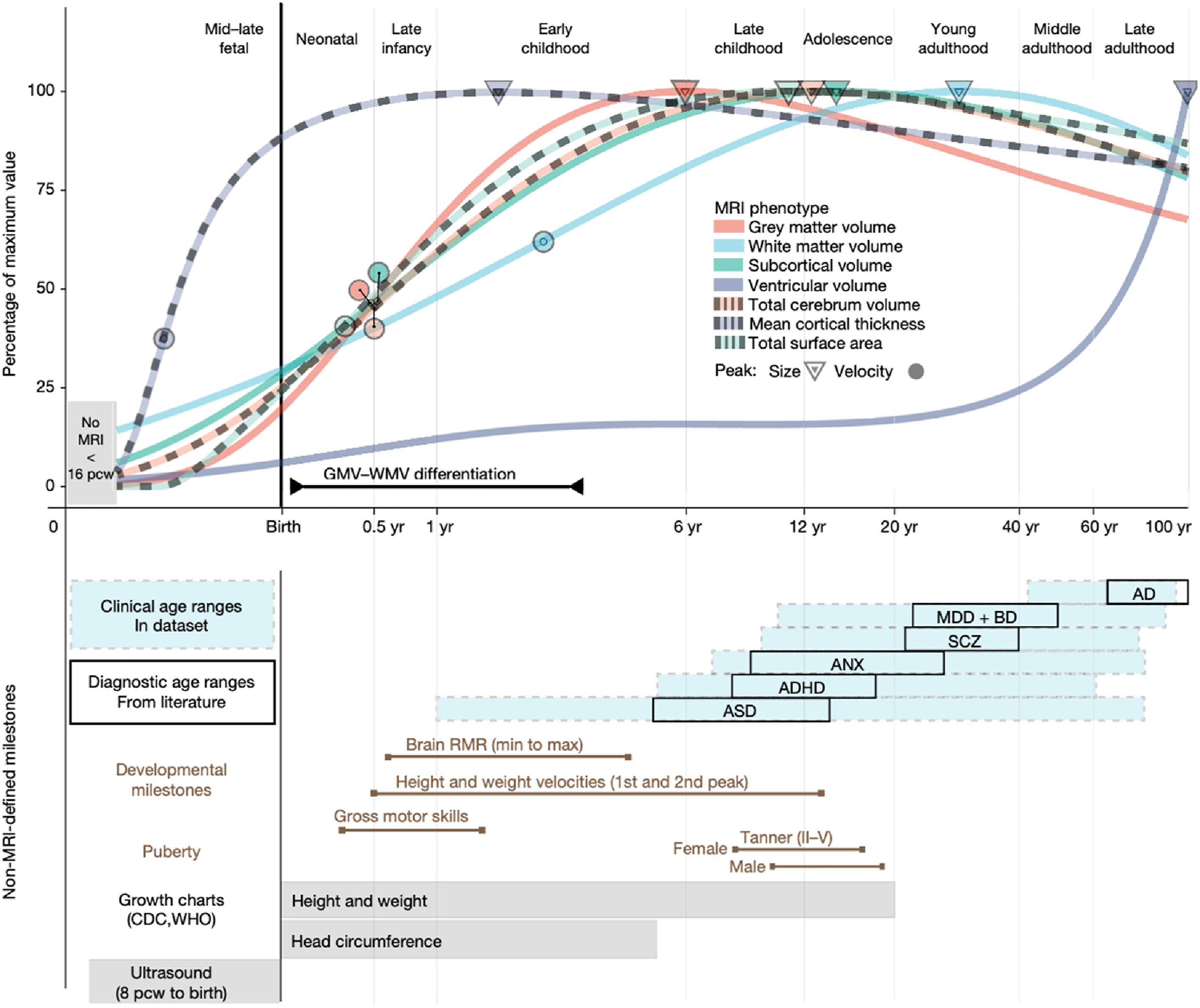
Subcortical GMV follows an intermediate growth pattern compared with GMV and WMV. It increases markedly from birth to around 5 years, stabilizes or slightly increases in childhood, peaks in adolescence, and slightly decreases thereafter. The striatum and cerebellar cortex volumes decrease linearly with aging, while other structures such as the amygdala, pallidum, thalamus, ventral diencephalon, and brainstem show an initial increase followed by a decrease with peak age of volume (32, 38, 28, 33 52, respectively). Fujita and colleagues reported an age-related subcortical GMV decrease without subdividing brain regions. Overall, GMV declines in healthy aging are notable but nonuniform, with regions like the prefrontal cortex, hippocampus, and temporal lobes showing greater volume reduction compared with the parietal and occipital lobes. These regions are crucial for cognitive functions such as memory, decision-making, and executive functions. Certain areas, such as the precuneus and inferior temporal region, are better preserved in physiologic aging compared with pathologic aging.
White Matter
Microstructural deterioration of the brain’s connective pathways through processes like axonal demyelination reduces information transfer efficiency leading to cortical disconnection which has a major role in aging of WM. , DWI, a quantitative method utilizing the Brownian motion of water molecules, enables the assessment of the underlying microstructure of brain WM.
White matter hyperintensity (WMH), best identified on T2WI, is associated with pathologic disease states such as multiple sclerosis, Alzheimer’s disease (AD), subcortical ischemic vascular dementia, and dementia with Lewy bodies but is also largely a part of normal brain aging. , WMH associated with normal aging appears on T2WI as periventricular and subcortical WM lesions characterized by: (1) hyperintensity on T2WI without contrast enhancement, (2) a lack of mass effect, and (3) no relation to infection, inflammation, or neoplasm. However, Haller and colleagues found that T2WI/FLAIR overestimates periventricular and perivascular lesions compared with histopathology, reporting more WMH than histopathologically confirmed demyelination.
Regarding global structural changes, WMV on T1WI generally decreases with age. WMV increases rapidly from birth to early childhood and continues increasing until early adulthood. According to Giorgio and colleagues and Bethlehem and colleagues, it begins to decline in mid-adulthood (40–60 years) and continues to decrease progressively with age ( Figs. 1 and 2 ). , Studies have indicated that the rate of WM atrophy varies across different brain regions. For instance, the frontal cortex and the corpus callosum exhibit more pronounced volume losses compared with other regions. These regional differences in atrophy may be linked to the varied functional impacts observed in cognitive aging, such as earlier declines in executive function and memory rather than vision.
Another sequence used to assess WM connectivity is DTI, which constructs brain fiber tracts by mapping the direction and magnitude of water diffusion in brain tissue. By measuring the anisotropic movement of water molecules, which is more restricted along the direction of axonal fibers, and applying diffusion-sensitizing gradients in multiple directions, DTI generates a tensor that describes the diffusion in each voxel, allowing for the reconstruction of fiber tracts through a process known as tractography. , Key findings in aging studies include a decline in fractional anisotropy (FA) and increases in mean diffusivity (MD) (ie, radial diffusivity and axial diffusivity) across different age groups. Changes in these metrics vary according to brain region. , Generally, reductions in FA are modest in early adulthood but increase over time, with the maximum effect noted in the prefrontal areas, correlating with cognitive decline and reduced executive function. Changes affecting the forceps minor (genu) of the corpus callosum are associated with reduced visuospatial performance and poor performance in tasks assessing perceptual reasoning and problem-solving. Another study correlated hearing loss in aging with decreased FA in the temporal inferior fronto-occipital fasciculus (IFOF). This correlates with increases in diffusivity metrics observed in the bilateral IFOF. MD also appears to be preferentially affected in the anterior brain region, as indicated by early microstructural changes. Overall, DTI metrics reveal widespread reductions in FA and significant increases in diffusivity measures, impacting memory and executive functions.
Because the conventional DTI axial diffusivity depends on MD in mathematical equations, conditions such as crossing fibers in the aging brain may produce faulty results. Therefore, different methods are being utilized, such as diffusion kurtosis imaging (DKI). While DTI provides valuable information about the diffusion of water molecules in tissues, it assumes a Gaussian distribution, which is not always true in biological tissue. DKI, on the other hand, accounts for non-Gaussian diffusion, capturing more complex microstructural characteristics of tissues and enabling the detection of subtle changes in brain structure and pathology that might be missed with DTI. , DKI parameters, such as mean kurtosis and radial kurtosis, have shown stronger correlations with developmental markers compared to DTI parameters like FA and MD.
Functional Magnetic Resonance Imaging
fMR imaging approximates brain activity by detecting changes in blood flow, leveraging the blood oxygenation level-dependent signal. This technique is based on increased oxygen consumption in active brain areas, leading to localized changes in blood flow that can be detected and mapped according to the magnetic state of hemoglobin. This allows for the visualization of brain activity in response to tasks or at rest.
fMR imaging studies have demonstrated that normal aging is associated with changes in both resting-state and task-based brain connectivity. In rsfMR imaging, older adults show reduced connectivity within the default mode network (DMN), which is linked to cognitive functions such as memory and self-referential thought. It is important to note, however, that altered DMN connectivity is also noted in pathologic conditions, with reduced connectivity in AD, and increased connectivity in frontotemporal dementia. In tfMR imaging, older adults exhibit altered activation patterns, often requiring greater recruitment of additional brain regions to maintain performance levels in cognitive tasks, indicating compensatory mechanisms.
tfMR imaging studies have revealed significant changes in brain activation patterns among different age groups during normal aging. Research indicates that older adults tend to recruit additional frontal brain regions during various cognitive tasks, a phenomenon often interpreted as a compensatory mechanism for age-related decline in other areas of the brain. For example, during episodic memory tasks, older adults exhibit increased bilateral frontal cortex activation compared with younger adults, who typically show more lateralized activation patterns. This shift toward bilateral activation in older adults is consistent with the Hemispheric Asymmetry Reduction in Older Adults (HAROLD) model.
Additionally, Davis and colleagues reported that older adults exhibit a Posterior-Anterior Shift in Aging (PASA), where there is a decrease in occipital (posterior) activation and an increase in frontal (anterior) activation during tasks involving visual attention, working memory, and episodic memory. However, Morcom and colleagues argued that these changes are nonspecific rather than compensatory.
Positron emission tomography
PET imaging has proven to be a powerful and noninvasive means of assessing the physiology of the aging brain. Unlike structural imaging modalities, PET can detect molecular changes such as glucose metabolism and proteinopathies. , CT and MR imaging can underlay PET images when acquired simultaneously to create hybrid imaging modalities as well. PET/CT has been the mainstay modality for PET hybrid imaging, where the effectiveness of localizing functional data has greatly improved diagnostic accuracy and has led to PET/CT being incorporated into clinical practice.
PET is unique in capturing various biological processes given its ability to measure the spatial accumulation of any radioactively tagged biologically active molecule. Various PET radiotracers having been used to study brain aging and related diseases in aging population including: 2-deoxy-2-[18F]fluoro- d -glucose ([ 18 F]FDG) used to capture glucose metabolism; 7-(6-(18F)fluoranylpyridin-3-yl)-5H-pyrido[4,3-b]indole ([ 18 F]Flortaucipir), 2-(1E,3 E)-4-(6-([11]C-methylamino)pyridin-3-yl)buta-1,3-dienyl ([ 11 C]PBB3), and [18F](S)−6-(3-Fluoro-2-hydroxypropoxy)−2-(N-methyl-2-aminopyridin-5-yl)quinoline ([ 18 F]THK-5351) mainly used in measuring tau deposition , ; 2-(1-{6-[(2-[18F]fluoroethyl) (methyl) amino]-2-naphthyl} ethylidene) malononitrile ([ 18 F]FDDNP) used in assessing neurofibrillary tangles and tau protein accumulation; 2-(4′-[11C]methylaminophenyl)-6-hydroxybenzothiazole ([ 11 C]PiB), 2-[3-(18F)fluoranyl-4-(methylamino)phenyl]-1,3-benzothiazol-6-ol ([ 18 F]Flutemetamol), and 4-[(E)-2-[6-[2-[2-(2-(18F)fluoranylethoxy)ethoxy]ethoxy]pyridin-3-yl]ethenyl]-N-methylaniline (18F-Florbetapir) used to analyze beta-amyloid plaques; 6-[18F]-L-fluoro-L-3, 4-dihydroxyphenylalanine ([ 18 F]FDOPA) to capture dopamine neurotransmitter activity ; and [ 15 O]-water and [ 15 O]-oxygen ([ 15 O]H 2 O and [ 15 O]O 2 ) in quantifying blood perfusion and oxygen metabolism ( Table 2 ).
[ 18 F]FDG-PET
While many radiotracers are used for PET imaging, the most important and widely utilized is [ 18 F]FDG, which captures glucose metabolism. [ 18 F]FDG is a glucose analog labeled with Fluorine-18 that accumulates in metabolically active cells after injection into the bloodstream. Inside these cells, FDG is phosphorylated by the enzyme hexokinase to form FDG-6-phosphate. Unlike glucose-6-phosphate, which is a substrate for phosphohexose isomerase, FDG-6-phosphate cannot proceed further down the glycolytic pathway and thus becomes trapped within the cell. The radioisotope then undergoes positron emission decay, generating high-energy gamma rays traceable by PET. As cerebral glucose metabolism is a key aspect of neuronal activity, [ 18 F]FDG plays a crucial role in assessing brain aging in a metabolic context.
[ 18 F]FDG PET’s sensitivity to metabolic changes has been extensively used to study healthy aging populations in contrast to those with neurodegenerative diseases such as AD. Specifically, decreased metabolism in the temporal and parietal lobes, posterior cingulate cortex, and precuneus gyrus has been linked with AD and mild cognitive impairment. Additionally, early detection of Parkinson’s disease through hypometabolism in the basal ganglia and detection of Lewy body dementias through hypometabolism in the occipital cortex have been documented.
Nevertheless, areas of the brain such as the frontal and temporal lobes and cingulate gyrus also show a progressive decrease in glucose metabolism during normal aging. Specifically, a decline in the cerebral metabolic rate of glucose (CMR GLC ) has been consistently observed in older adults across many large regions. For instance, Nugent and colleagues found a 7% lower CMR GLC in older adults compared with younger adults ( n = 44) across the whole brain. Deery and colleagues ( n = 620) corroborated this finding with pooled effect sizes showing significantly lower CMR GLC in older adults for the whole brain. Tumeh and colleagues described a 38% decrease in whole brain metabolism with age ( n = 81).
Regionally, the frontal lobe shows significant declines in glucose metabolism, with activation likelihood estimates identifying clusters of lower metabolism in the inferior frontal gyrus. , Similarly, the temporal, parietal, and occipital lobes show significant declines in glucose metabolism. , Notably, regions like the insula and anterior cingulate cortex, associated with cognitive and emotional processing, show significant metabolic declines. , , , Nugent and colleagues also noted lower metabolism in the putamen and thalamus, responsible for motor control and sensory signal relay, respectively ( Table 3 ) ( Figs. 3–5 ).
Age Group (Years) | Global Uptake (SUVmean) | Regional Uptake | Key Findings | Study |
---|---|---|---|---|
Neonates | ∼30% of adult rates | Low in frontal lobe, high in parietal, temporal, cerebellar, primary visual cortices, basal ganglia | Increasing metabolism correlates with developing sensorimotor functions and behaviors | Kennedy et al, 1957 |
0–4 | Rapid increase, surpasses adult rates by age 3 | High in parietal, temporal, cerebellar, visual cortices | Frontal lobe remains low until cognitive abilities develop | Tumeh et al, 2007; Kennedy et al, 1957 |
4–9 | Plateau at ∼1.3 times adult rates | High in parietal, temporal, cerebellar, visual cortices | Sustained high metabolism correlates with ongoing brain development | Tumeh et al, 2007; Kennedy et al, 1957 |
10–19 | Declines to adult rates by end of second decade | Decreasing in visual cortex | Reflects mature development of visual system and overall brain | Chugani et al, 1992 |
20–79 | Gradual decline | Significant decline in frontal, parietal, temporal, occipital lobes; preserved in basal ganglia, thalami, hippocampi, cerebellum, visual cortices | Increased cerebellum-to-cortex ratio, anterior-posterior gradient, hypometabolism in lateral orbital gyrus | Tumeh et al, 2007; Subtirelu et al, 2023, Deery et al, 2023, Nugent et al, 2014. |
78+ | ∼26% decrease compared with age 18 | Decrease in frontal, somatosensory areas | Global decrease in brain metabolism, marked hypometabolism in frontal lobe | Tumeh et al, 2007, Kuhl et al, Deery et al, 2023, Nugent et al, 2014 |
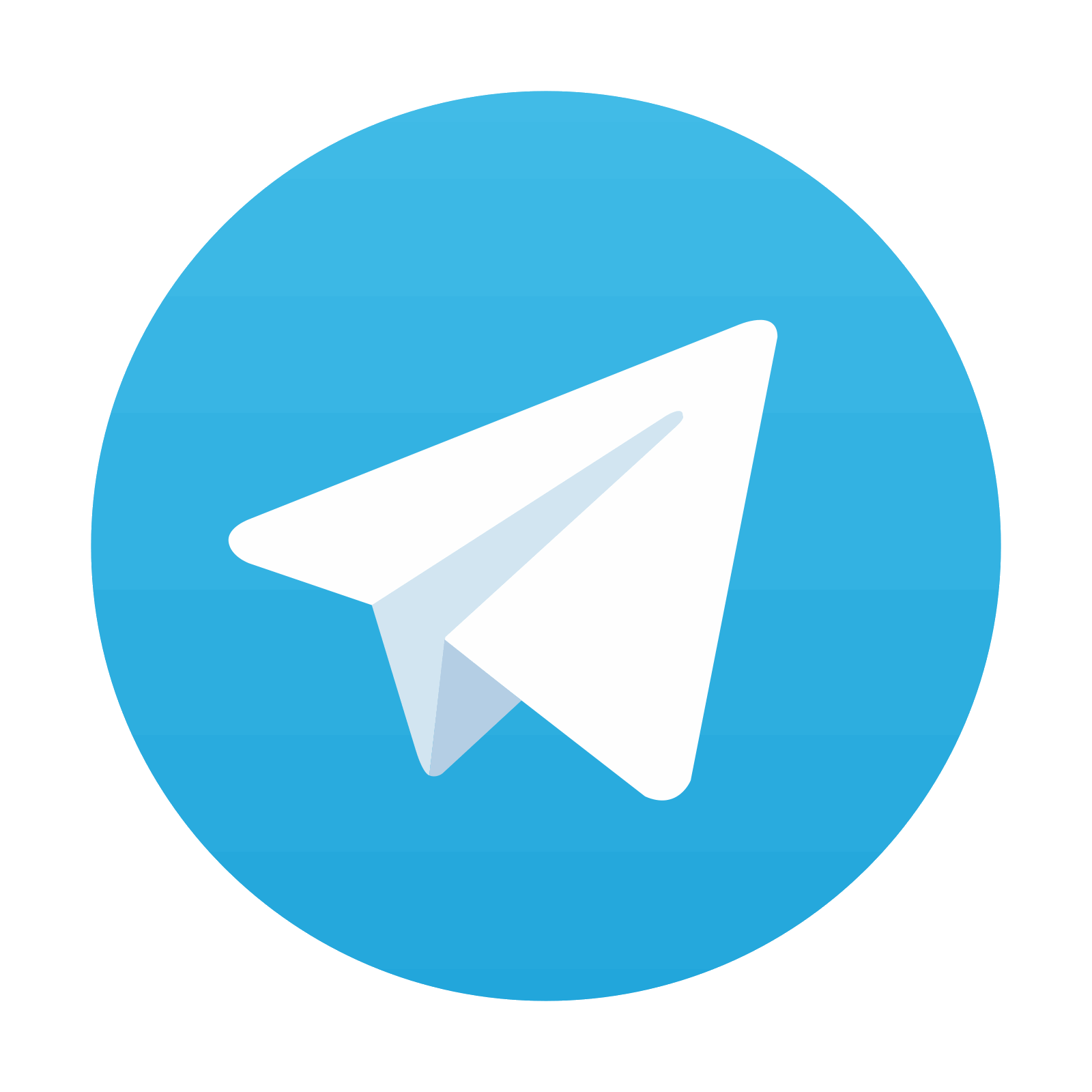
Stay updated, free articles. Join our Telegram channel
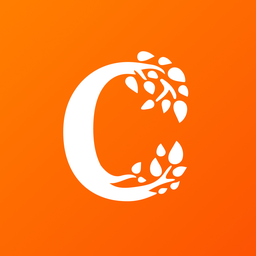
Full access? Get Clinical Tree
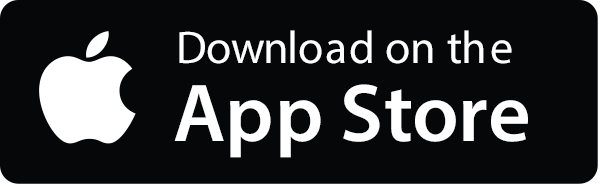
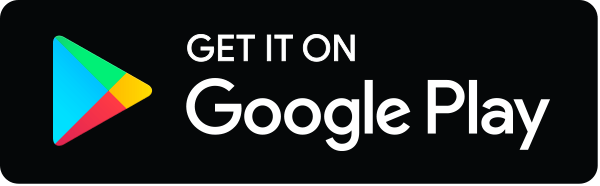
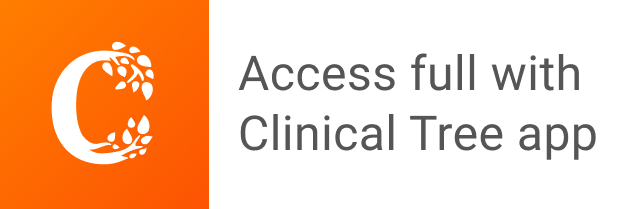