Introduction
Intensity-modulated proton therapy (IMPT) using pencil beam scanning (PBS) technology has revolutionized the practice of particle therapy in recent years. , In PBS delivery, a particle pencil beam is magnetically scanned in the plane transverse to the beam direction, creating a large field without requiring scattering elements in the beam paths. Monoenergetic pencil beams with different energies from an accelerator are stacked to create the desired dose distribution along the beam direction. , Neither an aperture nor a compensator is required, although external collimation could be beneficial for reducing the penumbra of low-energy beams.
The desired dose distribution over the clinical target volumes can be achieved by optimizing the weights of individual pencil beams with different energies by using an inverse planning process. Two general approaches have been used to optimize a scanning beam plan. The first is called multifield optimization (MFO), which simultaneously optimizes all spots from all fields. The second approach is single-field optimization (SFO), , in which each field is optimized individually to deliver a fraction of the prescribed dose to the entire target volume(s). The single-field uniform dose (SFUD) , , , is the most common application of SFO to produce a uniform dose over the entire target volume by each field. The single-field integrated boost (SFIB) is another application of the SFO technique reported by Zhu et al. The SFIB technique optimizes the plan with dose constraints to create the desired coverage of different target volumes by different dose levels. Clinically, MFO and SFO techniques have been applied to various disease sites. IMPT is one of the most advanced forms of radiation therapy available to date. Despite its superior dosimetric advantages, various challenges limit the realization of its full potential. Various technologies have been advanced and will continue to be advanced to meet these challenges.
Accelerator and beam delivery technologies
Traditionally, the most common configuration of a proton therapy facility consists of an accelerator with three to five treatment rooms. The accelerator, either cyclotron or synchrotron, has standard sizes and requires a fairly large room to host it. The standard gantry is also large and can weigh as much as 200 tons. Superconducting-based cyclotrons and compact synchrotrons have become available commercially in recent years that allow smaller-footprint accelerator rooms. All proton therapy system vendors offer single-room configurations at this time. These efforts have reduced the cost and made proton systems more affordable to more clinics. One vendor is offering a superconducting gantry, which not only reduces the weight of the gantry but also opens up more space for new and innovative imaging technologies to provide better imaging guidance. We expect that proton therapy delivery systems will be further miniaturized in the coming years.
The most common delivery method for PBS is spot scanning, which is a discrete step-and-shoot approach to beam delivery. The major advantage of the approach is that it is safe and reliable for delivering each spot. The main disadvantage is that it is less efficient owing to the “dead time” between the spots. Raster scanning is similar to spot scanning except that the beam is not switched off when moving to the next spot. , , This causes a small amount of transient dose that is delivered during the move. The transient dose should be included and modeled correctly by the treatment planning system (TPS). This technique is faster than spot scanning for treatment delivery because the dead time is reduced to the time required to travel to the next position. In continuous line scanning, the beam is continuously scanned, normally along the axes in the plane of constant energy. , The beam is switched off only when the energy is changed. Continuous line scanning is technically more challenging than either spot scanning or raster scanning, but it can deliver a beam very quickly and can be useful for repainting. Although spot scanning technology is the current standard in delivery technology, raster scanning (also called dose-driven continuous scanning) and continuous line scanning are becoming available commercially. These technologies improve the delivery efficiency and make layering and volumetric repainting more practical for motion mitigation.
Delivery of IMPT using PBS technology requires a switch to a different, usually lower, energy after completing a given energy layer. The energy switch time has been reduced for cyclotron- and synchrotron-based systems in recent years. For synchrotrons, the typical time required to change energy is on the order of 1 to 2 seconds. , This is the time required to deaccelerate the remaining protons with the previous energy in the synchrotrons and accelerate a new spill of protons with the new desired energy. The time needed for the adjustment of the beam transport line is similar between the two types of accelerator. For cyclotrons, the additional time is determined by how fast the energy selection system can be adjusted for the next energy. The energy change can be as fast as less than 0.1 second when an energy selection system is used, although most systems in clinical use would take approximately 1 to 2 seconds. For synchrotrons, the energy switching time is expected to be reduced significantly with a new technology called “multiple energy extraction,” which is currently being developed and implemented for commercial accelerator systems.
Proton arc therapy was proposed by Sandison et al. to reduce the lung dose relative to electron arc therapy. For men with prostate cancer, Rechner et al. , showed that the risk of second cancers using proton arc therapy is less or equal to that of photon volumetric modulated arc therapy (VMAT). Seco et al. proposed that proton arc therapy could reduce the range uncertainty effects and spare the healthy lung relative to photon VMAT for lung stereo tactic body radiation therapy. Proton arc therapy using spot scanning could be promising for further improving the dosimetric results for patients with locally advanced stage nonsmall cell lung cancer. We expect that the advantages of proton arc therapy will be further explored for possible clinical advantages in the near future.
Range uncertainty
Although it can be accurately measured in water, the proton beam range in a patient has an uncertainty, typically 3.5% of the range in current clinical practices. , Without any physiological variation, the range uncertainty in patients originates from (1) uncertainties in the Hounsfield units (HUs) of computed tomography (CT) images, (2) the conversion of HUs to proton stopping powers, and (3) the uncertainty of the stopping powers primarily from the mean excitation energy ( I -value). These uncertainties have the potential to alter the proton range and dose distribution, which would result in underdosing the target volume or overdosing critical structures. Additional uncertainties from setup could result in a combined effect of range uncertainties in inhomogenous tissues of a patient, which can also alter the ranges of protons and influence dose distribution. Range uncertainty is one of the fundamental physical limitations of particle therapy. Research efforts to directly reduce the range uncertainties and minimize the impact of range uncertainty are described further below.
Reduce the range uncertainty . Dual-energy CT (DECT) using kV x-rays has the potential to reduce uncertainties in stopping power ratios (SPRs) and therefore the uncertainties in the proton beam ranges over the single-energy CT (SECT). The DECT approach combines information from two images with different x-ray energies to resolve the ambiguities in the HUs-to-SPR conversion. Although it is generally agreed that there are improvements in determining SPRs with DECT over SECT, the reported reduction of uncertainties of SPRs varies from study to study, ranging from approximately 0.4% to 1.5%. , , One of the reasons preventing DECT from gaining larger benefits over SECT is high-level noise on DECT images. Comprehensive uncertainty analysis by Li et al. showed that the DECT method could reduce the overall range uncertainty to approximately 2.2% (2σ) in clinical settings.
Proton radiography (pRG) and proton CT (pCT) , provide direct measurements of proton SPRs and therefore more accurate estimates of proton beam ranges. pRG would allow direct range verification as well as image guidance. pCT does not suffer from metal artifacts as do kV x-ray CT images. However, many technical challenges remain to be solved before pCT imaging becomes routine practice in proton clinics around the world. One of the fundamental limitations is lateral straggling of the proton beam, which limits the spatial resolution of pRG and pCT and also results in image artifacts. One promising design is proton tracking, which is best able to handle the problem of multiple scattering of protons for pRG and pCT. ,
Minimize the impact of range uncertainty . One effective method to minimize the impact of range uncertainty is the use of robust optimization that incorporates setup and range uncertainties. , The robustness of a treatment plan can be assessed by using robust evaluation. A more difficult question is: What is clinically acceptable robustness? In an attempt to answer this question, Li et al. studied a group of patients with oropharynx cancer who received IMPT for possible correlation between local control and the robustness of the target volume coverage. ,
Monitoring the proton beam range in patients . In vivo monitoring of the proton beam range in patients would provide direct information about where the proton beam is stopped. Methods being developed include proton-induced positron-emission tomography, prompt gamma, , and proton-induced acoustics. ,
Treatment plans and dose calculations
The quality of an IMPT treatment plan depends on an effective planning process, particularly a good optimization. The current optimization process with commercial TPSs involves an iterative process to refine the optimization parameters. It is a trial-and-error process that can be time-consuming. Automatic treatment planning and optimization based on prior knowledge, machine learning, and artificial intelligence have the potential to generate the best possible plan for each patient.
Accuracy of dose calculation is critical to clinical implementation of IMPT. It was suggested recently that analytical dose calculation algorithms may not have sufficient accuracy for highly inhomogenous tissues such as lung. Monte Carlo simulation–based dose calculation is more desirable.
Motion interplay effect
When PBS technology is used to treat moving tumors, the dynamic pencil beam and target motion can lead to interference caused by similarities in the time scale involved in beam delivery and target motion; in other words, the motion of the target can result in misplacement of the individual pencil beam spots. This phenomenon, also referred to as the motion interplay effect, can cause additional degradation of the delivered dose distribution, potentially manifesting as extreme local tumor underdosage and normal structure overdosage. , A guideline for treating moving targets recently published by the Particle Therapy Co-Operative Group Thoracic Subcommittee recommends assessing the motion for each patient and using different motion mitigation strategies, including beam angle and scanning direction selection, four-dimensional (4D) robust optimization, layer or volume repainting, breath hold and gating based on the motion analysis, and 4D dynamic doses, as necessary.
Biological uncertainties
The relative biological effectiveness (RBE) of protons is defined as the ratio of the absorbed doses that produce the same biological effect or clinical end point between a high-energy photon and proton beam irradiation under the same conditions. Proton therapy treatments are currently planned assuming a constant proton RBE relative to high-energy photons of 1.1. The actual RBE of protons is a complex function of numerous factors such as treatment technique, dose, depth, cell type, oxygenation, intrinsic radiosensitivity, and the biological or clinical end point of interest. Different RBE models may predict different results. Linear energy transfer (LET) may in fact be a better surrogate for biological effects because it is a physical quantity, independent of models. By optimizing the plan such that the higher LET is in the target volume and the lower LET is in the critical normal structures, it may be possible to improve the tumor control probability and reduce the normal tissue complication probability (NTCP).
Variation of anatomy
An important source of uncertainty comes from changes in the patient’s anatomy, including weight loss, tumor shrinkage, and opening or blocking airways, which can significantly change the proton stopping power distribution along the path of the beam. The influence of these uncertainties becomes more significant in IMPT plans because the individual fields can be highly modulated, and steep dose gradients can be located in the middle of the target. A recent retrospective study showed that robust optimization using both original planning CT and adaptive CT image sets could improve the IMPT plan’s robustness with respect to anatomic changes in eight patients with lung cancer. By including synthetic CTs with varying nasal cavity filling in their robust optimization, van de Water et al. concluded that anatomic robust optimization effectively accounted for changes in nasal cavity filling during IMPT, providing substantially improved clinical target volume and organs-at-risk doses compared with conventional SFUD optimization.
Online adaptive planning for each fraction will be the ultimate adaptive strategy in radiation oncology. Recently, Bernatowicz et al. studied the feasibility of online IMPT adaptation by using fast, automatic, and robust dose restoration. The essential requirements for online adaptation include diagnostic-quality images with the patient in the treatment position for every fraction, automatic contouring, fast optimization, dose calculation, and online quality assurance for each adaptive plan. With recent advances in artificial intelligence and machine learning and fast dose calculation and optimization, online adaptive planning for patients undergoing IMPT will be come practical in the near future.
Cone-beam CT (CBCT) equipment has become standard in modern proton gantries. CBCT installed in proton treatment rooms typically has flat panels mounted on the gantry and x-ray tubes installed on the floor. A new design of the CBCT system involves its to the treatment couch with a ring. In general, CBCT image quality suffers from scatter and results in CT numbers that are not sufficiently accurate for proton dose calculation. One approach to improve the CT numbers of CBCT is to deform the planning CT scan onto the CBCT scan. , Alternatively, some proton therapy centers have installed CT-on-rails, which has diagnostic image quality and DECT capability. Integrating a magnetic resonance imaging scanner with proton treatment delivery is also being investigated by researchers and manufacturers.
Response of proton therapy
The superior physical dose distributions of IMPT have shown promising clinical advantages in some clinical scenarios. For example, an analysis with 50:100 (1:2) matching of IMPT to IMRT for patients with oropharyngeal carcinoma found that that IMPT was associated with reduced rates of feeding tube dependency and severe weight loss. The oncologic, toxicity, and functional outcomes after IMPT for such patients are encouraging. In a series of patients treated with IMPT for definitive reirradiation of thoracic cancers, Ho et al. concluded that that IMPT provided durable local control with minimal toxicity and suggested that higher doses may improve outcomes.
NTCP and tumor control probability after radiation therapy are multifactorial and complex functions of physical, biological, and clinical conditions. Machine learning has promise for navigating the complex nature of the problem. Applications of machine learning and artificial intelligence , could provide stronger evidence of the clinical advantages of IMPT.
Summary
IMPT is the most advanced radiation therapy modality available to date. With reduced physical and biological uncertainties, advanced imaging guidance, online adaptation, and better understanding of the outcomes, the full potential of IMPT can be realized in the near future.
References
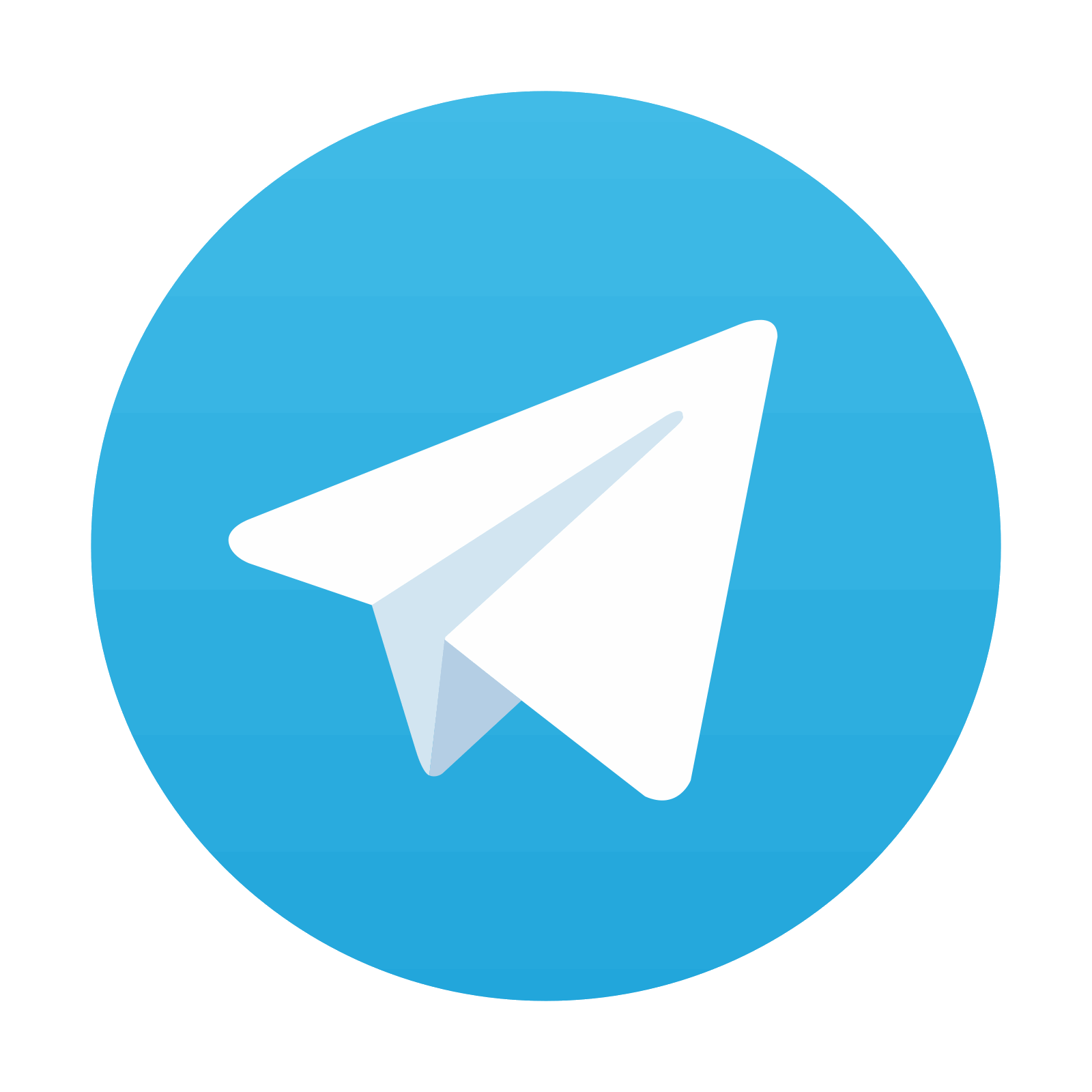
Stay updated, free articles. Join our Telegram channel
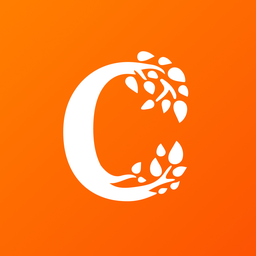
Full access? Get Clinical Tree
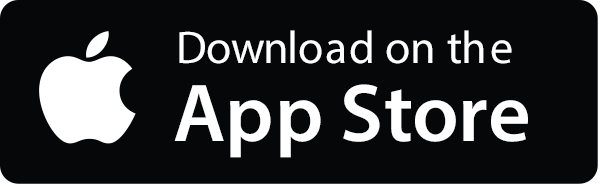
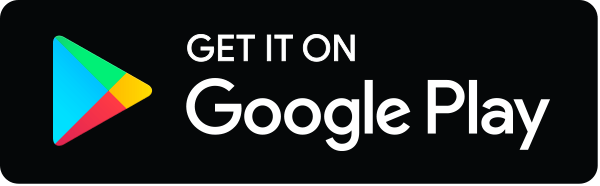
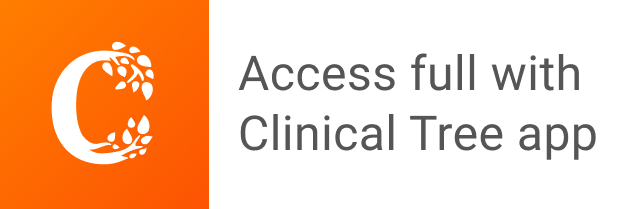