Abstract
Type 2 diabetes (T2D) is a persistent illness that has a high incidence rate. Still, there is no conclusive evidence on effectively improving blood sugar levels in patients through physical therapy. This study examined the regulatory effects of different intensities of low-intensity pulsed ultrasound (LIPUS) on T2D by stimulating brown adipose tissue (BAT). Eight-week-old C57BL/6J mice were divided into six groups (n = 10 per group): Control sham (C-Sham), Control-LIPUS (C-LIPUS), T2D-sham (T2D-Sham), T2D groups treated with LIPUS at spatial average-temporal-average intensity ( I sata ) of 60mW/cm² (T2D-L-60), 80mW/cm² (T2D-L-80), and 100mW/cm² (T2D-L-100). T2D models were induced by intraperitoneal injection of 40 mg/kg streptozotocin (STZ) three times after 12 wks of high-fat diet (HFD). The T2D-LIPUS group received LIPUS stimulation for 20 minutes per day for 6 weeks. The LIPUS stimulation had a duty cycle of 20%, a frequency of 1 MHz, and I sata of 60mW/cm², 80mW/cm², 100mW/cm². Subsequently, glucose tolerance tests (GTT) and insulin tolerance tests (ITT) were performed, and body fat content in mice was analyzed using nuclear magnetic resonance (NMR). Metabolic changes were monitored using metabolic cages. The results indicated that 80mW/cm² intensity level significantly improved glucose tolerance, insulin sensitivity, and metabolic function after LIPUS exposure. Significant reductions in body fat content and enhanced thermogenesis were observed, highlighting the potential of LIPUS in T2D management. This provides the basis for the dose study of LIPUS in the treatment of T2D.
Introduction
Diabetes is a metabolic disorder characterized by high blood sugar due to insulin dysfunction, leading to tissue damage over time. Type 2 diabetes (T2D) is a long-term metabolic disorder marked by persistent insulin resistance, posing a substantial risk of complications [ ]. T2D presents a significant and urgent public health challenge globally, reflecting a widespread metabolic disorder in modern society. As of recent data, the prevalence of T2D in China has significantly increased. According to the latest estimates, about 141 million adults in China are living with diabetes, which represents approximately 11.2% of the adult population [ , ]. The prevalence of T2D is rapidly increasing worldwide, affecting approximately 463 million adults and is anticipated to rise to 700 million by 2045 [ ]. Its onset is also increasingly observed in children and adolescents [ , ]. The progressive nature of T2D, associated with sedentary lifestyles, genetic predisposition, and dietary habits leads to metabolic derangements, resulting in hyperglycemia and an array of complications including retinopathy, neuropathy, nephropathy, and cardiovascular disease [ ].
Current treatment strategies for T2D typically include lifestyle modifications, oral antidiabetic agents and injectable insulin. Emerging classes, such as SGLT-2 inhibitors agonists and GLP-1 receptor, have demonstrated significant efficacy in lowering blood glucose levels and mitigating cardiovascular risks [ ]. For instance, sulfonylureas and thiazolidinediones, commonly prescribed oral agents, can result in weight gain and increase the risk of hypoglycemia [ ]. Moreover, extended use of metformin, a cornerstone of T2D therapy, may precipitate gastrointestinal disturbances like diarrhea and nausea [ ]. However, it is imperative to acknowledge the inherent limitations and potential adverse effects associated with these treatments, which may be constrained by potential side effects and variable efficacy [ , ]. Concerns regarding the effectiveness, cost, and long-term safety of current pharmacologic therapies highlight the need for innovative therapeutic strategies beyond pharmacological interventions [ ].
Low-intensity pulsed ultrasound (LIPUS) is a mechanic stimulation therapeutic modality that has been extensively studied for its potential applications in various medical fields [ ]. It operates by delivering acoustic energy in the form of pulsed waves, penetrating soft tissues without causing thermal damage [ ]. One of the primary mechanisms is the ability of LIPUS to induce mechanical stimulation at the cellular level, which can lead to the activation of signaling cascades and the upregulation of various growth factors and cytokines [ ]. This mechanical stimulation, in turn, can enhance cell proliferation, differentiation, and extracellular matrix production, all of which are critical for tissue repair and regeneration. Research on varying LIPUS intensity therapy has primarily focused on investigating its effects at varying intensities on bone healing, fracture repair, and osteoporosis management, providing valuable insights into optimal parameters for clinical application [ ]. Sun et al.’s [ ] study on LIPUS intensities ranging from 15 to 150 mW/cm² demonstrated a strong correlation with bone mineral density, bone microstructure, and mechanical strength, underscoring the importance of intensity as a critical factor in achieving therapeutic effects. Studies increasingly utilizes LIPUS in diabetic mouse models to target various metabolic factors and conditions, demonstrating promising outcomes for glycemic control and improved metabolic parameters on examining the specific metabolic effects of LIPUS, including insulin sensitivity, glucose metabolism, and adipose tissue function [ ]. Xu et al.[ ] indicated that LIPUS stimulated on visceral adipocytes could modulate obesity by activating the p38 MAPK pathway. This activation led to inhibited white adipocyte proliferation, increased apoptosis, and suppressed differentiation of white adipocytes [ ]. Study by Cao et al.[ ] demonstrated that LIPUS can activate the metabolic function of adipose tissue to improve glucose metabolism in obese mice. Furthermore, Nishida et al.[ ] revealed that LIPUS may inhibit lipid formation by altering the actin cytoskeleton and suppressing insulin signaling pathways. In addition, He et al.[ ]’s study on LIPUS stimulation of epididymal white adipose tissue (WAT) demonstrated that LIPUS alleviates obesity-induced insulin resistance, supporting broader findings that mechanical stimulation from LIPUS can modulate glucose metabolism. These results suggest that LIPUS stimulation of metabolic organs, such as adipose tissue, may influence metabolic functions and inflammation, impacting key glucose-regulatory processes by modifying insulin signaling pathways and reducing oxidative stress in tissues. T2D, like obesity, is also a metabolic disease, primarily characterized by disturbances in glucose and lipid metabolism, as well as insulin resistance. Therefore, LIPUS was used to treat type 2 diabetes.
Brown adipose tissue (BAT), often referred to as “brown fat” due to its characteristic color, a metabolically active tissue that plays a pivotal role in energy expenditure and glucose metabolism [ , ]. These studies suggest that BAT serves as a significant thermogenic organ and is recognized as an effective target for insulin sensitivity [ , ]. Unlike WAT, BAT stands out for its high mitochondrial content and the presence of uncoupling protein-1 (UCP1), enabling it to convert stored energy directly into heat, making it an attractive focus for metabolic interventions aimed at combating obesity and related disorders [ , ]. These studies highlight the role of BAT in regulating glucose and lipid metabolism, as well as insulin sensitivity. Using LIPUS to stimulate BAT could potentially noninvasively and without side effects activate BAT’s metabolic function to regulate T2D, addressing the growing global burden of metabolic diseases.
Therefore, this study aims to expand the current research on LIPUS by exploring its therapeutic effects on T2D. Specifically, we explored the impact of LIPUS on insulin sensitivity, glucose homeostasis, and related physiological parameters in a preclinical model of T2D. We utilized a diverse experimental device equipped with self-developed LIPUS technology. Employing a range of intensities (60mW/cm 2 , 80mW/cm 2 , 100mW/cm 2 ), we assessed the potential of LIPUS as a noninvasive and innovative approach to T2D management. The regulatory effects of these intensity levels on T2D mice were investigated by targeting the stimulation of BAT. T2D model mice were established through STZ injections and HFD. To evaluate the regulatory impact of LIPUS treatment, we compared the therapeutic effects of each intensity by conducting glucose tolerance tests (GTT), insulin tolerance tests (ITT), nuclear magnetic resonance (NMR) analysis, metabolic cage analysis, serum analysis, hematoxylin and eosin (H&E) staining, and qRT-PCR methods.
Materials and methods
Animals
Sixty male 4-week-old C57BL/6J mice (initial body weight: 20.16 ± 1.06 g) were obtained from the Institute of Developmental Biology and Molecular Medicine at Fudan University. Mice were housed in standard-sized cages with ventilation racks, maintained in a pathogen-free, controlled environment at a temperature of 23 ± 1°C and humidity of 60 ± 5%. They followed a 12-h light-dark cycle (from 8 a.m. to 8 p.m.) and had ad libitum access to food and water. The diet consisted of either a standard rodent chow diet or a HFD (D12492) comprising 60% kcal from fat, 20% from protein, and 20% from carbohydrates, primarily in the form of saturated fat. All procedures were approved by the Animal Ethical Committee of Fudan University and followed the guidelines set forth in the “Guide for the Care and Use of Laboratory Animals” published by the National Institutes of Health.
T2D was induced in mice through the administration of a low-dose streptozotocin (STZ) injection following a 5-wk HFD regimen. In the 5th wk of the HFD intervention, mice received intraperitoneal injections of STZ at a dose of 40 mg/kg body weight [ , , ] or an equivalent dose of citrate buffer solution over consecutive three d. Freshly prepared STZ and citrate buffer solution were administered daily. After the STZ injection, fasting blood glucose (FBG) levels were measured using the OneTouch Verio Reflect™ Blood Glucose Monitoring System. This method aligns with Wang et al.’s study, which characterizes T2D by elevated blood glucose levels and reduced insulin sensitivity, as confirmed through insulin tolerance testing (ITT) [ ].
Mice were divided into six groups (n = 10 per group), with an equal number of mice in each group: Control mice (C-Sham), control mice with sham LIPUS exposure (C-LIPUS), T2D mice with sham LIPUS exposure (T2D-Sham), T2D groups treated with LIPUS at different spatial average-temporal-average intensity ( I sata ) , that is 60 mW/cm² (T2D-L-60), 80 mW/cm² (T2D-L-80), and 100 mW/cm² (T2D-L-100). During the group allocation process, body weight and blood glucose levels were carefully balanced across all groups.
LIPUS treatment
The mice groups received LIPUS stimulation for 20 mins per session, five days a wk, over a period of six wks with a frequency of 1 MHz, a duty cycle of 20%, and I sata levels of 60 mW/cm², 80 mW/cm², and 100 mW/cm² respectively. The C-LIPUS group underwent a similar procedure with ultrasound that was not activated. The LIPUS was generated using a custom ultrasonic therapy device ( Fig. 1a ) developed by the Intelligent Medical Ultrasound Lab at Fudan University (Shanghai, China) [ , ]. Ultrasound parameters, including duty cycle, frequency, PRF and treatment duration, were manually adjusted to specific levels (duty cycle of 20%, frequency of 1.5 MHz, PRF of 1.0 kHz and daily exposure duration of 20 mins). Intensity levels were modulated during the exposure and calibrated at the beginning and throughout the experiment using a high-resolution (1 mW), fast-response (<3 s) ultrasound power meter (UPM-DT-1000 PA, Ohmic Instruments, MO, USA). Mice were depilated and then continuously anesthetized using continuous inhalation of isoflurane to maintain a horizontal prone position. The targeted region for LIPUS therapy BAT in mice, located in the interscapular back neck area [ ], was exposed to a 25-mm diameter transducer (Suzhou, China), with ultrasonic coupling gel applied between the skin and the circular transducer to ensure optimal contact and transmission of ultrasound waves. The entire experimental process is schematically illustrated in the timeline depicted in Figure 1b .
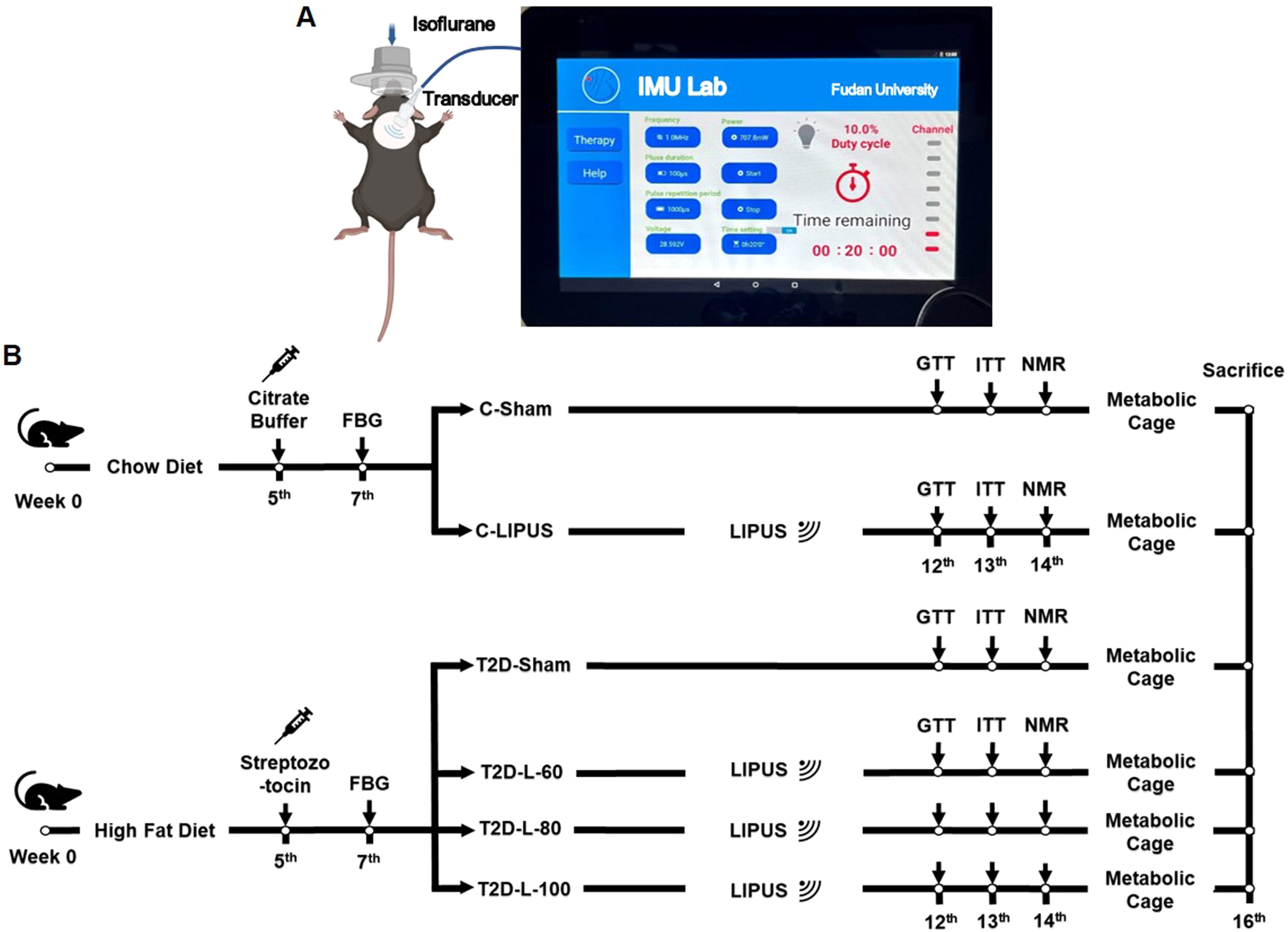
Glucose and insulin tolerance test
To assess glucose uptake and insulin resistance, we performed intraperitoneal GTT and intraperitoneal ITT five wks following LIPUS exposure. Mice underwent a 12-h fasting period before the intraperitoneal GTT with an administration of 1 g/kg glucose. The ITT with an administration of 0.5 unit/kg insulin was conducted, requiring a 6-h fasting period from 9 am to 3 pm for the mice. Blood samples were collected through a small incision in the tail, and blood glucose concentrations were measured at intervals of 30, 60, 90, and 120 minutes after the intraperitoneal injections. Results were subsequently determined using the area under the curve (AUC) method.
NMR of body composition
To assess the impact of LIPUS on the body composition of the mice, measurements were taken for fat mass and free liquid composition using Time Domain NMR technology with the Bruker LF50H TD-NMR minispecTM. The analyzer is 70 cm wide and 110 cm deep. Measurements are radiation-free (no special shielding is needed in requirement), rapid, completely silent, and offer a highly accurate and user-friendly method for assessing fat content in mice without anesthesia. Raw NMR data were processed using Bruker software to calculate body composition parameters, including fat mass and lean mass, among others.
Metabolic cage
To evaluate the impact of LIPUS on metabolic function, a 72-h metabolic cage test was conducted after 4 wks of LIPUS exposure. The Promethion Metabolic Cage System from Sable (Nevada, USA) was utilized to monitor individual mice’s oxygen consumption, carbon dioxide production, water intake, food intake and activity over 72 h. The metabolic exchange rate is determined by the ratio of carbon dioxide production to oxygen consumption.
Serum biochemical analysis
Mouse blood extracted at sacrifice was analyzed for total cholesterol (T-Cho) and triglyceride (TG), tumor necrosis factor-alpha (TNF-α) and Interleukin-1β (IL-1β). Following blood collection, samples were promptly centrifuged at 3500 rpm for 15 minutes at 4°C to separate the serum. The serum was then stored at -80°C until further biochemical analysis. Serum T-cho and TG in serum were measured following the manufacturer’s instructions (A111-1-1, A110-1-1, Nanjing Jiancheng Bioengineering Institute, China). Serum insulin (90080, Ultra-Sensitive, USA), TNF-α (MEC1003, Anogen, Canada), and IL-1β (MEC1010, Anogen, Canada) were quantified using enzyme-linked immunosorbent assay (ELISA) kits according to the manufacturer instructions.
Histologic analysis
After sacrifice, two sides of epididymal white adipose tissue (eWAT), subcutaneous white adipose tissue (sWAT), and BAT from the interscapular and back neck areas were excised from the mice and weighed. BAT was divided into three pieces, and sWAT into two pieces. All of two pieces of SWAT, two pieces of BAT and eWAT were fixed in 4% paraformaldehyde for 24 hours, embedded in paraffin, and sectioned for H&E staining and UCP1 immunohistochemical (IHC) staining. The stained slides were analyzed using an optical microscope (Nikon Eclipse E100), and standard scanning images were generated. CaseViewer software (Version 2.4.0, 3D Histech, Budapest, Hungary) was utilized to review the scanned images, and ImageJ software (Version 1.53n, National Institutes of Health, USA) was employed to measure the diameter and area of adipocytes as well as the area of UCP1 expression.
Real-time quantitative PCR (qRT-PCR)
The last piece of BAT was snap-frozen in liquid nitrogen for PCR analysis. Then, the tissue was utilized for total RNA extraction, followed by reverse transcription to generate cDNA. Subsequently, qRT-PCR analysis was conducted to quantify the expression of proinflammatory cytokines, including TNF-α and IL-1β. Total RNA was isolated from adipose tissue samples with TRIzol (Thermo Fisher Scientific) according to the manufacturer’s instructions. The RNA concentration and purity were assessed using a NanoDrop spectrophotometer (Thermo Fisher Scientific). cDNA was synthesized from 1 µg of total RNA using the RR037A kit (Takara) in a total volume of 20 µL. qRT-PCR was carried out using the SYBR Green PCR Master Mix (TaKaRa, China) on an ABI 7900HT sequence detection system. The reaction mixture consisted of 10 µL SYBR Green Master Mix, 1 µL forward primer (10 µM), 1 µL reverse primer (10 µM), 2 µL cDNA template and 6 µL nuclease-free water, for a final volume of 20 µL. The thermal cycling conditions were as follows: initial denaturation at 95°C for 10 mins, followed by 40 cycles of 95°C for 15 s and 60°C for 1 min. Data were analyzed using the comparative Ct (ΔΔCt) method with GAPDH as the internal control. The primers used for qRT-PCR are listed in Table 1 .
Gene name | Forward primer sequence (5′–3′) | Reverse primer sequence (5′–3′) |
---|---|---|
TNF-α | AGCCCATGTTGTAGCAAACC | TGAGGTACAGGCCCTCTGAT |
IL-1β | GAAATGCCACCTTTTGACAGTG | TGGATGCTCTCATCAGGACAG |
GAPDH | AAGGTCGGAGTCAACGGATT | AGTGGATGCAGGGATGATGT |
Statical analysis
All results were presented as mean ± standard error of the mean (SEM). Data were analyzed using GraphPad Prism software (Version 8.3.0, San Diego, USA). Statistical comparisons between two groups were assessed using a two-taile d Student’s t-test, while differences among multiple groups were assessed using two-way analysis of variance (ANOVA) followed by posthoc Holm-Sidak multiple comparison tests.
Results
Establishment of T2D mice model
Following STZ and citrate buffer injection in the 5th wk of HFD, confirming the establishment of the T2D mice model involved checking the FBG levels, which exhibited a significant elevation compared to the chow-diet-fed mice ( p < 0.0001) ( Fig. 2a ). Simultaneously, there was a notable increase in the body weights of HFD-fed mice, reaching 34.55 ± 2.1 g, in contrast to the chow-diet-fed ( p < 0.0001) at 27.8 ± 1.2g ( Fig. 2b ). According to the protocol, mice with blood glucose levels exceeding 180 mg/dl (10.3 mmol/L) [ ], were selected to represent the established T2D model. When the mice were divided into six groups, weights were carefully balanced between the T2D-Sham group, T2D-L-60, T2D-L-80 and T2D-L-100 groups. These mice were designated as T2D mice and subsequently used as recipients for the LIPUS treatment experiments.
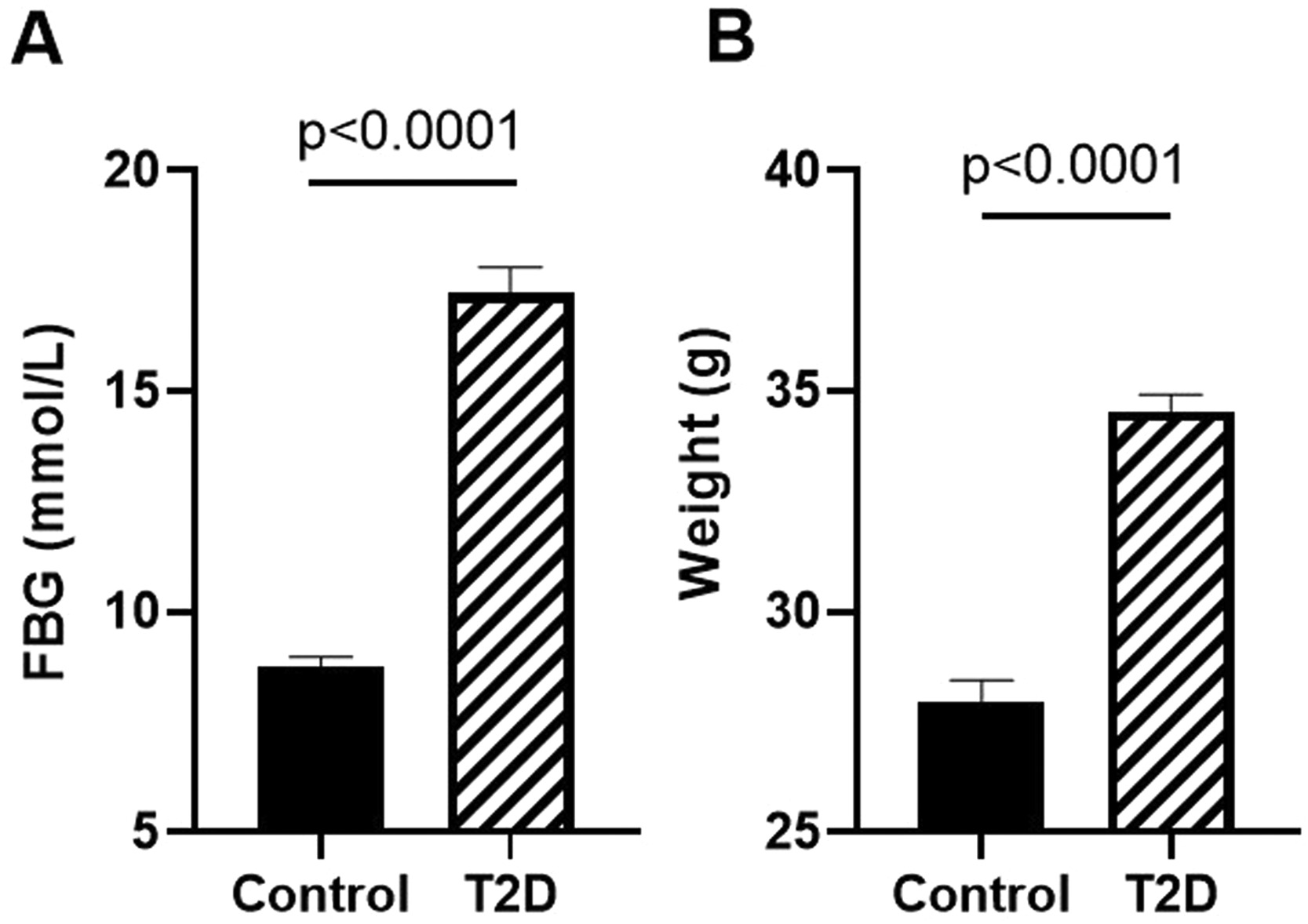
LIPUS alleviating insulin resistance in T2D mice
After a 4-wk exposure to LIPUS, GTT and ITT were conducted to assess insulin resistance across all experimental groups. In control groups, LIPUS treatment showed no significant effects in the C-LIPUS group ( p = 0.1229) ( Fig. 3a –f) compared to the C-Sham group. In comparison with C-Sham group, the T2D-Sham group exhibited substantial impairment in glucose tolerance ( p < 0.0001), as evidenced by the intraperitoneal GTT ( Fig. 3a ) and the corresponding AUC ( Fig. 3b ). Additionally, reduced insulin sensitivity was observed in the T2D-Sham group mice ( p < 0.0001), as indicated by the ITT ( Fig. 3d ) and the AUC of the ITT ( Fig. 3e ).
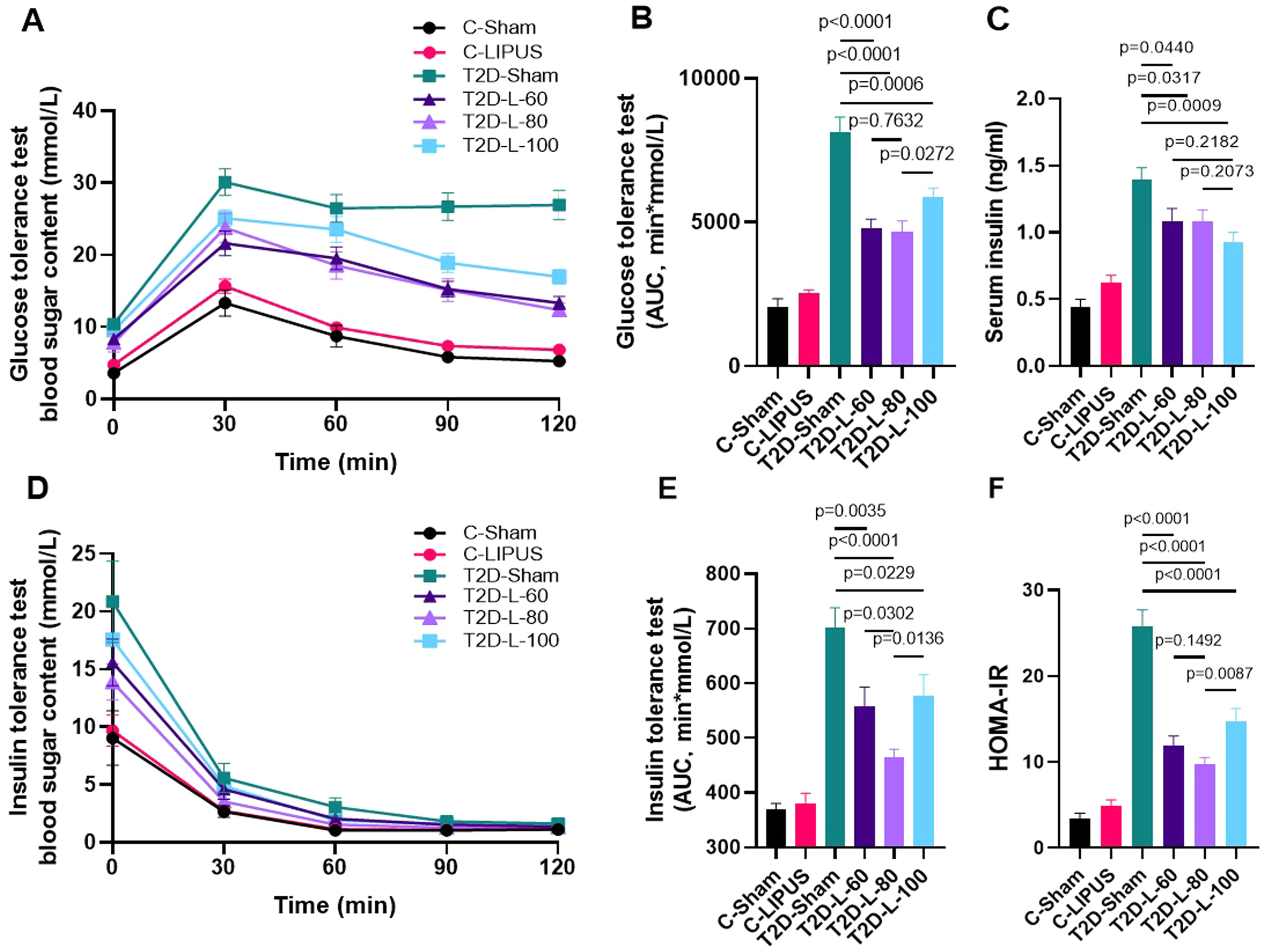
Overall, mice treated with LIPUS at intensities of 60, 80 and 100 mW/cm² demonstrated enhanced glucose tolerance or improved insulin resistance as opposed to the T2D-Sham group ( p < 0.0001, p < 0.0001 and p = 0.0006, respectively), as evidenced by both GTT and ITT outcomes ( Fig. 3a , 3b , 3d , 3e ). Notably, the T2D-L-60 and T2D-L-80 groups exhibited superior tolerance in GTT compared to the T2D-L-100 group ( p = 0.0287 and p = 0.0272) ( Fig. 3a , 3b ). In addition, the T2D-L-80 group demonstrated significantly enhanced insulin tolerance compared to the other two treatment groups T2D-L-60 ( p = 0.0302) and T2D-L-100 ( p = 0.0136) ( Fig. 3d , 3e ). Moreover, the serum insulin levels of the T2D-L-100 group exhibited lower insulin levels compared to the T2D-L-60 ( p = 0.0282) and exhibited lower trends compared to T2D-L-80 ( p = 0.2073) groups ( Fig. 3c ). Homeostatic Model Assessment of Insulin Resistance (HOMA-IR) analyses unveiled that all the treatment groups exhibited lower insulin resistance levels compared to the T2D-Sham group ( p < 0.0001) ( Fig. 3f ). Among the treatment groups, the T2D-L-80 group demonstrated trend toward reduced insulin resistance levels compared to the T2D-L-60 group ( p = 0.1492) and was significantly lower than the T2D-L-100 group ( p = 0.0087) ( Fig. 3c , 3f ).
LIPUS reduces body weight, fat content and lipid levels
NMR body composition analysis and blood lipid levels revealed that the C-Sham and C-LIPUS groups exhibited no significant differences in serum TG and T-Cho concentrations ( Fig. 4c , 4d ). Conversely, the T2D-Sham group mice demonstrated significantly higher obesity and blood lipid levels as opposed to the control groups ( p < 0.0001) ( Fig. 4c , d). Overall, the T2D-L-60 (mean weight = 29.8364), T2D-L-80 (mean weight = 29.47), and T2D-L-100 (mean weight = 29.8) groups showed weight loss compared to the T2D-Sham group ( p = 0.0031, p = 0.0038, and p = 0.0024) ( Fig. 4 a) and a decrease in fat content ( p = 0.0002, p = 0.0002, and p < 0.0001) ( Fig. 4b ). Additionally, mice treated with LIPUS exhibited a significant reduction in fat content in both sWAT ( p < 0.0001) and eWAT ( p < 0.0001) ( Fig. 4f , 4g ) compared to the untreated group. T2D-L-80 and T2D-L-100 groups displayed showed a trend toward reduced serum TG concentration compared to the T2D-Sham group mice ( p = 0.0900 and p = 0.1797 respectively) ( Fig. 4c ). Additionally, compared to the T2D-Sham group, T2D-L-60 and T2D-L-100 groups exhibited significant smaller BAT mass ( p = 0.0466 and p = 0.0213, respectively), T2D-L-80 group displayed a tendency to lower BAT mass ( p = 0.3150) ( Fig. 4e ). All treatment groups showed a trend toward reduced serum T-Cho concentration ( Fig. 4d ) ( p = 0.6724, p = 0.5445 and p = 0.2219, respectively) ( Fig. 4d , 4e ). Notably, the T2D-L-80 group mice revealed a tendency towards lower body weight ( Fig. 4a ) but higher fat mass ( Fig. 4b ) with a greater proportion of BAT content relative to WAT content relative to the T2D-L-60 ( p = 0.6319, p = 0.4972) and T2D-L-100 groups ( p = 0.5576, p = 0.3442) ( Fig. 4e , 4f ). Furthermore, we did not observe any significant difference in food intake between T2D mice and those receiving LIPUS treatment ( Fig. 4h ).
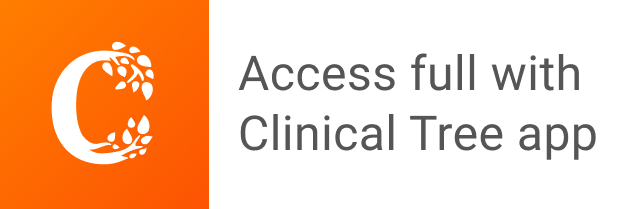