(1)
U.S. Army Research Institute of Environmental Medicine, Natick, MA, USA
(2)
Department of Pharmacology, Rutgers University-Robert Wood Johnson Medical School, Piscataway, NJ, USA
Abstract
The imaging of spontaneously occurring tumors in mice poses many technical and logistical problems. Recently a mouse model was generated in which a chimeric protein consisting of HIF-1α oxygen-dependent degradation domain (ODD) fused to luciferase was ubiquitously expressed in all tissues. Hypoxic stress leads to the accumulation of ODD-luciferase in the tissues of this mouse model which can be identified by noninvasive bioluminescence measurement. Crossing this transgenic mouse with tumorigenic mice yields solid tumors with hypoxic cores that may be successfully imaged and characterized using the technique described herein.
Key words
ODD-luciferaseBioluminescenceIn-vivoHypoxiaTumorHIF1 Introduction
Since the mid twentieth century, when the discovery of X-radiation and its development as a diagnostic aid provided the first opportunity to noninvasively image a living being, the detection and evaluation of tumors in vivo have been an area of ongoing research. In the latter half of the twentieth century, a wide array of noninvasive, live-tissue imaging options were developed to complement and even replace traditional X-radiography [1]. Rapid development and improvement in our understanding of ultrasonic imaging [2], computed tomography [3], positron emission tomography [4], magnetic resonance imaging [5], and nuclear scintigraphy [6] has provided us with a host of new tools to observe and measure tumors in vivo. These techniques provide access to a realm of detailed and important information, but to properly obtain and interpret this information, we must rely upon the expertise of board-certified radiologists within the medical profession who possess extensive training and experience.
In the laboratory, efforts are constantly underway to improve our understanding of tumor biology. To this end, scientists have developed a variety of tools, including the use of immunocompromised mice as hosts for human xenografts, thus providing researchers a method with which they may observe the growth and development of human tumors within the complex biological milieu of a living animal. These models, while tremendously useful, also lack some key properties that would allow them to better represent the development of tumors in their natural environment [7]. For instance, often xenografts behave in a different manner when transplanted in mice than they would in their native host [8, 9].
The recognized deficiencies in xenograft models have resulted in efforts to develop improved murine tumor models and imaging systems. Advances in genetic manipulation provide us with the ability to selectively knockout tumor suppressor genes and to overexpress oncogenes, thus allowing scientists to generate tumors in specific tissues which mimic the genetic changes found in human cancers [9–13]. Although murine tumors, whether induced or naturally occurring, are not always perfect analogs of their human counterparts, they provide valuable information on tumor behavior. In addition, they can be excellent systems for determining the efficacy of many anti-cancer therapeutics. One example of murine tumor models successfully used to this end is the development of transgenic mice predisposed to the development of mammary tumors [11, 14, 15].
While the development of transgenic mice has provided us with excellent models of stochastic, spontaneous tumorigenesis and development in vivo, the noninvasive imaging techniques available to observe these tumors remain largely inadequate. Human imaging modalities, including ultrasonography, computed tomography, and magnetic resonance imaging, often pose technical, financial, and regulatory challenges to the typical scientist. As a result, the scientific community has come to rely on more “user friendly” alternatives, the most popular of which is the use of bioluminescent or fluorescent imaging. Many effective in vivo imaging models have now been developed which depend either upon the conditional co-expression of bioluminescent reporter genes with specific tumor-associated genes, or upon the use of fluorescent reporter molecules directed towards specific cancer-associated cell proteins [16]. These models, however, are inherently limited in use to a single type or subtype of cancer, and may not be broadly applied to tumors of differing origins.
With the model described here, we attempted to alleviate many of the deficiencies of murine mammary tumor models by combining the ease of use of bioluminescent imaging with the superior biological model of stochastically occurring in vivo tumors in immunocompetent mice. This method, as with all tumor imaging techniques, depended upon the differentiation of tumor cells from normal tissue. It has long been known that there are differences between cancerous tissue and normal tissue that, if exploited, might provide a foundation for a more broadly applicable tumor imaging system [17]. For instance, all solid tumors have been shown to experience a certain degree of relative hypoxia when compared with surrounding tissue [18–20].
Recently, a transgenic mouse expressing the oxygen-dependent degradation (ODD) domain of the Hypoxia Inducible Factor 1-α (HIF1-α) gene fused to a luciferase bioluminescent reporter gene was developed by Safran et al. [21]. This model allows for the bioluminescent imaging of regions in which hypoxia is present. The HIF1-α gene is ubiquitously transcribed and translated, but under normoxic conditions, the enzyme HIF prolyl hydroxylase utlilizes oxygen to hydroxylate the HIF ODD. The hydroxylated ODD then recruits von Hippel–Lindau protein (pVHL), a ubiquitin E3 ligase, leading to poly-ubiquitination of HIF1-α and its subsequent degradation by the proteasome. Conversely, under hypoxic conditions, lack of hydroxylation of the ODD domain leads to the accumulation of HIF1-α which acts as a transcriptional regulator for a number of hypoxia-induced genes. By universally expressing the ODD-luciferase gene in mice, Safran et al. developed an organism in which luciferase would be continuously transcribed and translated in all cells, but would also be rapidly degraded under normoxic conditions. Only under conditions of hypoxia can luciferase accumulate to the point that it can act upon the substrate luciferin and generate a bioluminescent signal that may be imaged.
Our technique is based upon the hypothesis that solid tumors should be visible in ODD-luciferase expressing mice due to the relative hypoxia experienced by the tumor cells, essentially allowing for the use of the ODD-luciferase transgenic model as a platform for noninvasive imaging of spontaneous solid tumors in mice [22]. For our work, we utilize mice predisposed to the development of mammary gland tumors (MMTV-neu and Beclin1 +/−). These mice were cross-bred with ODD-luciferase mice. The resultant cross-breeds yielded tumors which generated substantial bioluminescent signals clearly discernable from background tissue luminescence. This technique may be used to track tumor growth and development longitudinally over several weeks.
2 Materials
2.1 Anesthesia
1.
Compressed oxygen—provided either by tank or via central building supply.
2.
Isoflurane anesthetic (Forane) (Baxter Healthcare, Deerfield, Ill.)
3.
IVIS Spectrum II workstation (Caliper Life Sciences, Hopkinton, MA), with integrated oxygen flow meters, isoflurane vaporizer, and induction chamber.
4.
Tumor-bearing ODD-luciferase transgenic mice (ODD-luciferase mice are available from Jackson Labs, Bar Harbor, ME as FVB.129S6-Gt(ROSA)26Sor tm1(HIF1A/luc)Kael /J, stock number 006206) (see Note 1).
2.2 Luciferin Preparation and Injection
1.
VivoGlo Luciferin, In Vivo Grade (d-luciferin may also be used. All substrates hereafter will be referred to simply as “luciferin”).
2.
Sterile Phosphate Buffered Saline (PBS).
3.
500 ml sterilized glass beaker.
4.
Ice with container.
5.
Sterile 10 ml tubes.
6.
−20 °C Freezer.
7.
Sterile 60 cc Luer Lock syringe.
8.
22 μm syringe filter.
9.
Aseptic tissue culture hood, properly installed.
10.
Stock solution of 30 mg/ml luciferin in PBS, frozen.
11.
Sterile Phosphate Buffered Saline.
12.
Ice with container.
13.
Sterile 10 ml tubes.
14.
−20 °C Freezer.
15.
Sterile 10cc Luer Lock syringe.
16.
Aluminum foil.
17.
Aseptic tissue culture hood, properly installed.
18.
Gram scale (for weighing mice).
19.
Prepared solution of 3 mg/ml luciferin in sterile PBS, thawed on ice in light-shielded tube.
20.
Sterile 1 ml syringe.
21.
Calculator (if needed).
22.
26 g needle.
2.3 Initial Imaging
1.
IVIS Spectrum II in vivo imaging station (or equivalent) with LivingImage Software, version 3.2 or later (Caliper Life Sciences, Hopkinton, MA).
2.
Anesthetized tumor-bearing ODD-luciferase transgenic mice (ODD-luciferase mice are available from Jackson Labs, Bar Harbor, ME as FVB.129S6-Gt(ROSA)26Sor tm1(HIF1A/luc)Kael /J, stock number 006206).
2.4 Post-imaging Recovery
1.
Standard mouse cage with wire top.
2.
Heat source (heating pad, heat lamp, or other thermostatically controlled heat source).
2.5 Image Processing
1.
Windows-based personal computer.
2.
LivingImage software, version 3.2 or later (Caliper Life Sciences, Hopkinton, MA).
2.6 Depilation
1.
Depilatory cream of choice.
2.
Small animal electric hair clippers (battery or AC powered).
3.
Cotton towels, pledgets, towelettes, or “4 × 4” surgical sponges.
3 Methods
3.1 Anesthesia
For all imaging procedures, anesthesia was limited to inhalational isoflurane. Isoflurane is the preferred anesthetic for both induction and maintenance due to the reduced stress on the animal (see Note 1).
1.
Prepare IVIS imaging station for use. This included switching on the apparatus, waiting for the unit to self-calibrate, and ensuring that the heating pad within the imaging station is warm via both temperature read-out and tactile confirmation.
3.
Remove selected mice from cages and place the mice in an induction chamber connected to an isoflurane vaporizer.
4.
Turn on the oxygen and set the isolation chamber flow-meter at 1 L/min.
6.
Observe mice for signs of general anesthesia, notably cessation of movement and decreased respiratory rate (see Note 4).
7.
3.2 Luciferin Preparation and Injection
1.
Turn out or black out all nonessential light sources in the room. All procedures should be carried out in a darkened aseptic tissue culture hood (see Note 10).
2.
Place 330 ml of cold PBS, pH 12, in a 500 ml glass beaker.
3.
Take 10 mg of luciferin solid and dissolve in the 330 ml of sterile PBS, gently swirling to mix, to yield a solution of 30 mg/ml luciferin.
4.
Filter the 30 mg/ml solution by drawing the solution into a 60cc syringe and then forcing the solution through a 0.22 μm filter into sterile, pre-labelled 10 ml canonical tubes. Place tubes in a −20 °C freezer for storage.
5.
Turn out or black out all nonessential light sources in the work room. All procedures should be carried out in a darkened aseptic tissue culture hood (see Note 10).
6.
Take one 10 ml tube of 30 mg/ml luciferin from the −20 °C freezer and immediately wrap with aluminum foil to shield the solution from ultraviolet light.
7.
Thaw the 30 mg/ml luciferin by warming in your hands, then place on ice.
8.
Fill a 10 ml tube with 9 ml PBS.
9.
Draw 1 ml of 30 mg/ml luciferin solution by pipette or syringe, and add to the prepared tube of PBS to create a solution of 3 mg/ml luciferin in PBS.
10.
Cover the tube with aluminum foil, mix gently, and place on ice for immediate use.
11.
Return the 30 mg/ml luciferin stock to the −20 °C freezer for storage.
13.
Calculate the amount of luciferin needed for injection. In this protocol, a dose of 50 mg/kg was used (see Note 12).
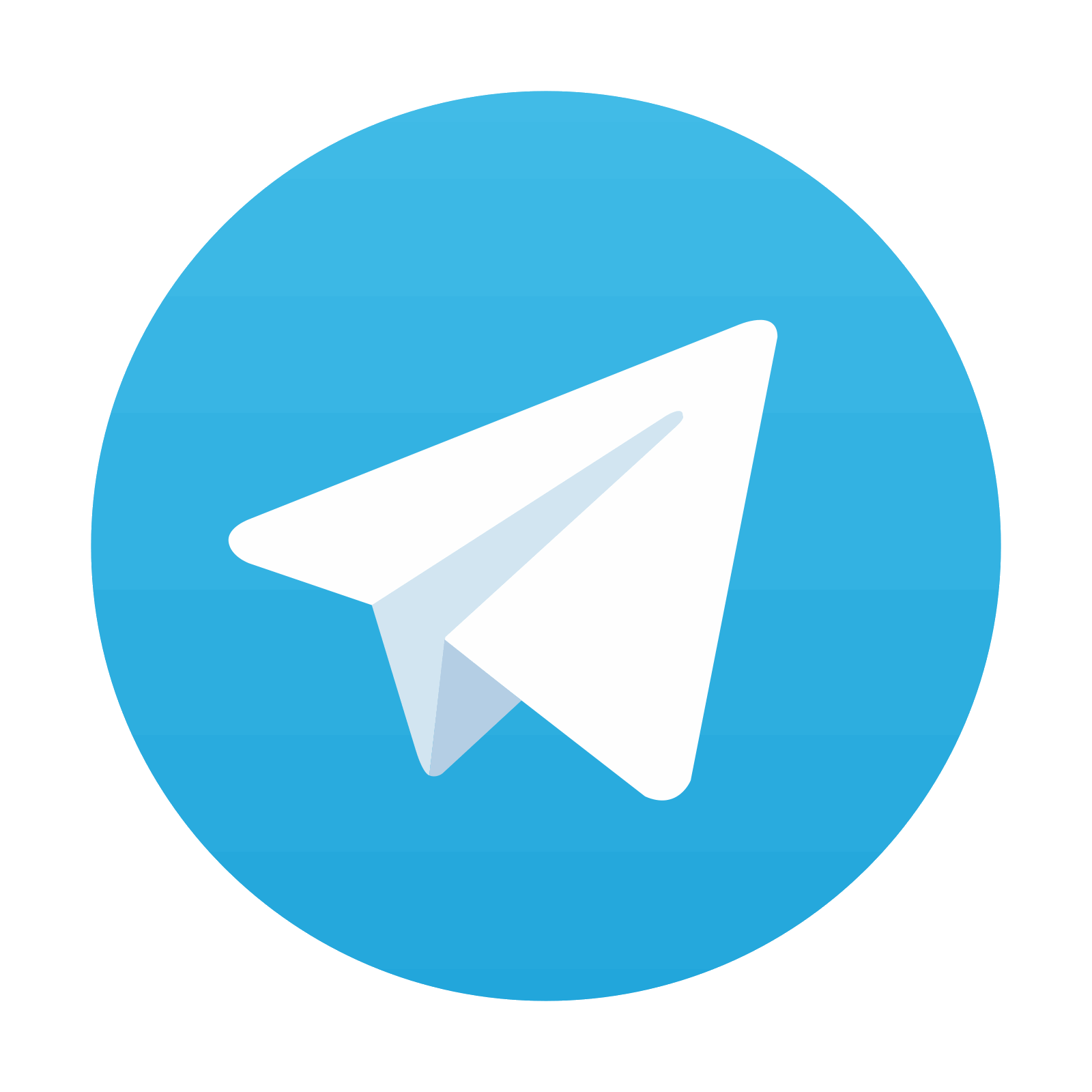
Stay updated, free articles. Join our Telegram channel
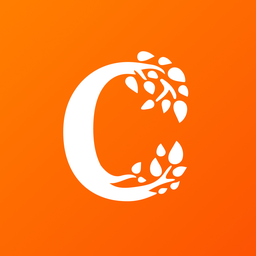
Full access? Get Clinical Tree
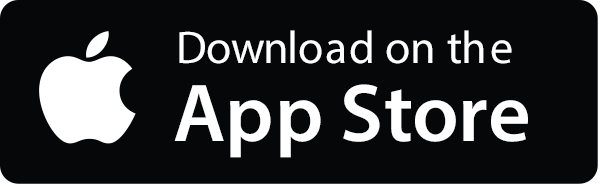
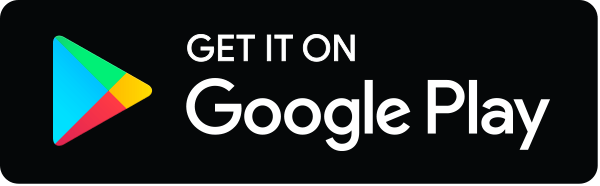
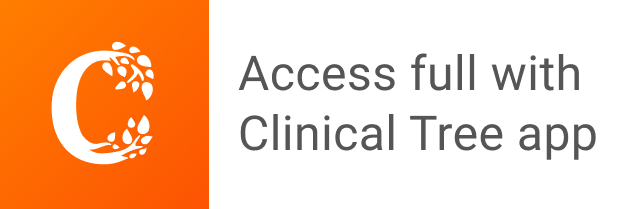