Interventional magnetic resonance (MR) neurography is a minimally invasive technique that affords targeting of small nerves in challenging areas of the human body for highly accurate nerve blocks and perineural injections. This cross-sectional technique uniquely combines high tissue contrast and high-spatial-resolution anatomic detail, which enables the precise identification and selective targeting of peripheral nerves, accurate needle guidance and navigation of the needle tip within the immediate vicinity of a nerve, as well as direct visualization of the injected drug for the assessment of appropriate drug distribution and documentation of the absence of spread to confounding nearby nerves.
Key points
- •
Interventional magnetic resonance (MR) neurography uniquely combines highest tissue contrast and high-spatial-resolution MR neurography with interventional MR.
- •
Interventional MR neurography affords accurate nerve blocks and perineural injections through direct visualization of nerve targets, accurate needle tip visualization, and objective assessment of the distribution of the injectant.
- •
Interventional MR neurography benefits patients and operators through the absence of procedure-related ionizing radiation.
- •
MR neurography-guided nerve blocks carry a high degree of validity because targets, needle tip, and distribution of injectants are visualized with high accuracy.
- •
Selective MR neurography-guided nerve blocks are especially helpful to identify neuropathies of the pudendal nerve, posterior femoral cutaneous nerve, lateral femoral cutaneous nerve, and obturator nerve.
Introduction
Interventional magnetic resonance (MR) neurography is a combination of high-contrast and high-spatial-resolution MR neurography and interventional MR imaging. MR neurography has the distinct ability to map the course of a nerve in its entirety from the spinal cord origin to the periphery and localize an abnormal appearance to a specific site. With interventional MR imaging, the nerve target and surrounding anatomic structures, as well as the location and spread of the injected drug, can be directly visualized. With use of proper technique, MR neurography-guided nerve blocks offer exquisite technical accuracy that provides the interventionalist with the means for an objective assessment of the technical adequacy of blocks and validity of pain responses.
In addition to history and physical examination, diagnostic MR neurography is used to rule out neoplastic neuropathies, identify abnormal nerves, and localize the site of impairment. On diagnostic MR neurography, signs of nonneoplastic neuropathy include enlargement of a nerve, abnormal T2 prolongation, effacement of the fascicular-like internal structure, irregularity and thickening of the mesoneurium, perineurium, and epineurium, impaired axonal flow, and perineural soft tissue scarring.
In concert with history, a skilled physical examination, targeted conductive nerve studies, and high-spatial-resolution diagnostic MR neurography, perineural injections are used to identify a pain-mediating nerve and treat neuropathic pain ( Table 1 ). Diagnostic nerve blocks with a local anesthetic are used to prove or disprove that a particular nerve is a substantial contributor to the patient’s pain syndrome. A nerve block may be used to confirm an MR neurographically abnormal-appearing nerve as the pain generator, to identify the pain-mediating nerves in the setting of multiple MR neurographically abnormal-appearing nerves, and to identify a pain-mediating nerve in the case of a normal-appearing MR neurography examination. Perineurally delivered corticosteroids may result in an interruption of inflammatory pathways on a cellular level and sustained pain relief.
Diagnostic Nerve-Related Injection | Therapeutic Nerve-Related Injections |
---|---|
Clinically suspected neuropathy | Adjunct to conservative treatment |
Discordance between clinical findings and imaging (false negative or false positive results) | Inoperable condition |
Presurgical testing (eg, neurolysis, neurotomy, and tumor resection in multiple lesions) | Bridging of prolonged recovery following surgery |
Unsatisfactory postsurgical results and recurrence | Induction of atrophy of a muscle causing nerve compression |
Allergy to iodinated contrast agents | Unsatisfactory postsurgical results and recurrence |
Small piriformis muscle not adequately visualized or targeted with other techniques | Contraindication or adverse effects to systemic pain medications/steroids and allergy to iodinated contrast agents |
MR neurography guidance is especially suited for deeply situated and small targets and for injectants that require accurate drug delivery, such as Botulinum neurotoxin. Interventional MR neurography rivals computed tomography because it provides superior contrast resolution and avoids potentially harmful ionizing radiation exposure. MR imaging guidance exemplarily complies with the ALARA (as low as reasonably achievable) practice mandate and is especially valuable in adolescents, young women of childbearing age, and pregnant women. Cumulative radiation doses can be avoided if serial nerve block protocols are used. It represents a preferred technique in cases where computed tomography, ultrasound, and radiograph fluoroscopy guidance is challenging or failed.
Factors that influence the availability of interventional MR neurography are local expertise, technical equipment, availability, cost related to the local health care environment, and time constraints and therefore may be currently limited to specialized centers. However, clinical 3 T wide-bore scanners have gained broad acceptance and equip many sites to perform MR neurography-guided procedures.
In this article, the technical background of interventional MR neurography is discussed, concepts and designs of nerve block regimens are reviewed, MR neurography-guided injection techniques are described, and targets and procedures for the diagnosis and therapy of nerve pain related to the pudendal nerve, posterior femoral cutaneous nerve, lateral femoral cutaneous nerve, and obturator nerve are illustrated.
Introduction
Interventional magnetic resonance (MR) neurography is a combination of high-contrast and high-spatial-resolution MR neurography and interventional MR imaging. MR neurography has the distinct ability to map the course of a nerve in its entirety from the spinal cord origin to the periphery and localize an abnormal appearance to a specific site. With interventional MR imaging, the nerve target and surrounding anatomic structures, as well as the location and spread of the injected drug, can be directly visualized. With use of proper technique, MR neurography-guided nerve blocks offer exquisite technical accuracy that provides the interventionalist with the means for an objective assessment of the technical adequacy of blocks and validity of pain responses.
In addition to history and physical examination, diagnostic MR neurography is used to rule out neoplastic neuropathies, identify abnormal nerves, and localize the site of impairment. On diagnostic MR neurography, signs of nonneoplastic neuropathy include enlargement of a nerve, abnormal T2 prolongation, effacement of the fascicular-like internal structure, irregularity and thickening of the mesoneurium, perineurium, and epineurium, impaired axonal flow, and perineural soft tissue scarring.
In concert with history, a skilled physical examination, targeted conductive nerve studies, and high-spatial-resolution diagnostic MR neurography, perineural injections are used to identify a pain-mediating nerve and treat neuropathic pain ( Table 1 ). Diagnostic nerve blocks with a local anesthetic are used to prove or disprove that a particular nerve is a substantial contributor to the patient’s pain syndrome. A nerve block may be used to confirm an MR neurographically abnormal-appearing nerve as the pain generator, to identify the pain-mediating nerves in the setting of multiple MR neurographically abnormal-appearing nerves, and to identify a pain-mediating nerve in the case of a normal-appearing MR neurography examination. Perineurally delivered corticosteroids may result in an interruption of inflammatory pathways on a cellular level and sustained pain relief.
Diagnostic Nerve-Related Injection | Therapeutic Nerve-Related Injections |
---|---|
Clinically suspected neuropathy | Adjunct to conservative treatment |
Discordance between clinical findings and imaging (false negative or false positive results) | Inoperable condition |
Presurgical testing (eg, neurolysis, neurotomy, and tumor resection in multiple lesions) | Bridging of prolonged recovery following surgery |
Unsatisfactory postsurgical results and recurrence | Induction of atrophy of a muscle causing nerve compression |
Allergy to iodinated contrast agents | Unsatisfactory postsurgical results and recurrence |
Small piriformis muscle not adequately visualized or targeted with other techniques | Contraindication or adverse effects to systemic pain medications/steroids and allergy to iodinated contrast agents |
MR neurography guidance is especially suited for deeply situated and small targets and for injectants that require accurate drug delivery, such as Botulinum neurotoxin. Interventional MR neurography rivals computed tomography because it provides superior contrast resolution and avoids potentially harmful ionizing radiation exposure. MR imaging guidance exemplarily complies with the ALARA (as low as reasonably achievable) practice mandate and is especially valuable in adolescents, young women of childbearing age, and pregnant women. Cumulative radiation doses can be avoided if serial nerve block protocols are used. It represents a preferred technique in cases where computed tomography, ultrasound, and radiograph fluoroscopy guidance is challenging or failed.
Factors that influence the availability of interventional MR neurography are local expertise, technical equipment, availability, cost related to the local health care environment, and time constraints and therefore may be currently limited to specialized centers. However, clinical 3 T wide-bore scanners have gained broad acceptance and equip many sites to perform MR neurography-guided procedures.
In this article, the technical background of interventional MR neurography is discussed, concepts and designs of nerve block regimens are reviewed, MR neurography-guided injection techniques are described, and targets and procedures for the diagnosis and therapy of nerve pain related to the pudendal nerve, posterior femoral cutaneous nerve, lateral femoral cutaneous nerve, and obturator nerve are illustrated.
Technique and equipment
Similar to the progression of interventional MR imaging from low-field strength to 1.5 T, the transition of interventional MR neurography from 1.5 T to 3 T yields similar benefits. Because of a near-linear relationship between the static magnetic field and spin polarization, 3 T field strength results in an approximately 1.8 to 2 times increase of the detectable MR signal, when compared with 1.5 T field strength. The higher MR signal afforded by a 3 T MR imaging system can be used for higher spatial image resolution and improved visualization of small nerves or for increased temporal resolution and faster MR imaging acquisition. In addition to the increase in signal-to-noise ratios, 3 T MR imaging also has the potential to generate increased contrast to noise ratios and better conspicuity of abnormal nerve. Because of an inverse square root relationship between the time needed for image acquisition and signal, images can be obtained up to 4 times faster at 3 T when compared with 1.5 T. Owing to the direct proportionality of the number of image acquisitions, the reduction of the number of excitations from 2 to 1 will decrease the length of time of image acquisition by 50%, thereby maintaining a net increase in signal-to-noise ratio of 40%. Wide-bore magnet designs can offer similarly sufficient patient access than dedicated open MR imaging systems. If the isocenter of the bore can be reached, table movement can be minimized and needle maneuvering can be performed while the patient is in the bore of the magnet.
The static magnetic field, radiofrequency pulses, and the gradient magnetic field contribute to special conditions of an interventional MR imaging environment. Similar to diagnostic MR imaging, an effective screening procedure for patients, operators, and other individuals entering the MR suite is crucial in order to guard the safety of MR interventions and to avoid accidents. In addition to general contraindications of MR imaging, pacemakers require careful consideration.
Devices that cannot be used safely in an MR environment may experience considerable traction forces that may be strong enough to accelerate an otherwise considered safe device to cause serious impact injuries or cause temperature increases of metallic and conductive objects, which can be high enough to cause burn injuries to patients and operators. A conventional stainless steel injection needle, for instance, may not be used safely for interventional MR neurography. A variety of MR-compatible needles and accessories are commercially available for different field strengths.
In order to ensure accurate and safe procedures at 3 T, several factors that may adversely affect device visualization and patient safety need to be considered. Specifically at 3 T, the increased precession frequency of protons requires radiofrequency excitation pulses with shorter wavelength and higher energy, resulting in higher energy transfers and depositions into the human body. Spin excitation may be performed with body coil techniques rather than surface transmit coils in order to limit local heating and in normal mode with a power deposition not to exceed 2 W/kg.
The power deposition of radiofrequency pulses increases with field strength, the square of the flip angle, and the number of radiofrequency pulses in a given time. The use of conventional fast spin-echo pulse sequences may require adjustment to different radiofrequency pulse types, shorter radiofrequency pulse durations, decreased flip angles, fewer slices, and different repetition times.
Gradient echo sequences have favorable specific absorption rate properties because of often small flip angles and lack of 180° refocusing pulses. However, the exaggerating effect of gradient echo sequences on local field inhomogeneities and susceptibility-related artifacts of even MR-conditional needles at lower field strengths may interfere with MR imaging guided injection procedures at 3 T field strength.
Despite modifications of pulse sequence protocols to limit energy deposition, heating of a metallic injection needle remains a possibility at 3 T. In addition to the radiofrequency-related increase in energy deposition, heating of injection needles may occur through electromagnetic resonance effects, for which an injection needle may become susceptible during interventional MR imaging at 3 T static field strength. The magnitude of this effect depends on the physical length of the injection needle and the radiofrequency wavelength. The injection needle may serve as an antenna for the electromagnetic field of the radiofrequency pulses and the induction of a current. The maximum effect theoretically occurs when the length of the needle matches half the length of the radiofrequency wave, which may be considered the critical length. At 0.2 T, the critical length is approximately 188 cm and decreases to approximately 23 cm at 1.5 T. When compared with 1.5-T static magnetic field strength, at 3 T, the radiofrequency wavelength shortens by 50% with an approximate radiofrequency wavelength of 26 cm in water. An injection needle of 13 cm length or longer placed in water-containing tissue might, therefore, act as a suitable antenna for a 126-MHz radiofrequency pulse. Needles exceeding lengths of 10 cm need to be used with caution and may require further pulse sequence adaptations.
The constant and reliable visualization of the injection needle is a prerequisite for effective and safe percutaneous MR-guided drug delivery. For MR neurography-guided injections, passive needle visualization technique is accurate, safe, and simple because no additional equipment or postprocessing of MR images is needed. The needle artifact is created by the lack of mobile protons of the metallic needle, localized spin dephasing, local gradient disturbances, and signal displacement in frequency-encoding directions. Optimized spin-echo pulse sequences can result in the display of the true needle position with an error margin of 1 mm at 1.5 T.
The needle artifact reaches a magnitude with needle orientations at 90° orientation to the static magnetic field, which is the case for procedures performed in the true transverse plane in a system with a horizontally oriented bore magnet. At 3 T, this technique is prone to an increased size of the resultant susceptibility-related needle artifact. In order to compensate for those effects, MR-compatible needles are typically manufactured from alloys of relatively low magnetic susceptibilities, such as titanium, nickel, cobalt, molybdenum, and chromium.
The type of pulse sequence and parameters, such as echo time, voxel size, readout, and radiofrequency bandwidth, further influence the needle artifact and enable the operator to optimize the appearance of the needle artifact. Although dephasing is less pronounced with turbo spin-echo sequences, short echo times can further decrease the needle artifact. Increasing the readout bandwidth minimizes signal displacement in in-plane frequency encoding direction and limits the size of the needle artifact. Maximizing the slice encoding gradient strengths minimizes through-plane displacement and the resulting needle artifact, which can be achieved through a smaller field-of-view and thin slice thickness. Needle optimization steps often come at the expense of a decreasing signal-to-noise ratio, which may require compensation by additional excitations.
Reliable visualization of the distribution of the injected agent and detection of spread to nearby structures is necessary to judge the technical success of a nerve block and the validity of a nonconfounded pain response. Injectants can be visualized based on their naturally long T2 constants. In case the needle is still in place, most injectants can be visualized by their high contrast-to-noise ratio to surrounding tissues with T2-weighted pulse sequences using echo times in excess of 100 ms. If fat suppression is desired, inversion recovery is the technique of choice because spectral fat saturation will fail because of the altered local static magnetic field strength around the needle tip. This technique can be of specific advantage in patients with hypersensitivity to iodine, for whom iodine-based contrast agents as used, such as computed tomography and radiographic fluoroscopy, are contraindicated.
The combined use of surface and table element coils in a sandwich configuration allows parallel imaging acquisition with an acceleration factor of at least 2. A 5-step workflow algorithm may be used ( Table 2 ). A large multichannel surface coil is advantageous for the acquisition of initial focused MR neurography images, whereas for needle placement, a smaller multichannel surface coil allows better access to the interventional site and sterile coverage.
Step | Action |
---|---|
1 | Focused MR neurography for visualization of the target and planning of the needle path |
2 | Determination of a suitable skin entry point using skin markers |
3 | Needle placement using intermittent MR imaging guidance |
4 | Confirmation of the final needle tip position |
5 | Optional sterile saline or sterile D5 water test injection followed by drug injection with monitoring of adequate distribution by the intermittent acquisition of fluid-sensitive MR images |
Versatile MR imaging pulse sequences for MR imaging-guided injection procedures at 3 T are listed in Table 3 . An initial high-spatial-resolution MR neurography sequence (see Table 3 , sequence 1) with an in-plane resolution of approximately 0.6 × 0.6 mm is used for visualization of the target nerve and planning of a safe needle path. Accurate needle visualization can be achieved with the use of high receiver bandwidth conventional turbo spin echo (see Table 3 , sequence 2) and half-Fourier acquisition single-shot turbo spin echo (see Table 3 , sequence 3) sequences, which differ in spatial resolution, edge sharpness, and acquisition speed. The distribution of the delivered injectant is in most cases efficiently visualized with a fast non-fat-saturated T2-weighted half-Fourier acquisition single-shot turbo spin echo sequence (see Table 3 , sequence 4). The addition of an inversion pulse for fat suppression causes a marked increase in fluid conspicuity (see Table 3 , sequence 5).
Number | 1 | 2 | 3 | 4 | 5 |
---|---|---|---|---|---|
Sequence type | Two-dimensional turbo spin echo | Two-dimensional turbo spin echo | Half-Fourier acquisition single-shot turbo spin-echo | Half-Fourier acquisition single-shot turbo spin-echo | Half-Fourier acquisition single-shot turbo spin-echo |
Weighting | Intermediate | Intermediate | T2 | T2 | T2 |
Orientation | Axial | Axial and sagittal | Axial and sagittal | Axial and sagittal | Axial and sagittal |
Repetition time (ms) | 7110 | 2500 | 2290 | 3440 | 3660 |
Echo time (ms) | 28 | 24 | 89 | 120 | 90 |
Inversion time (ms) | — | — | — | — | 220 |
Echo train length | 25 | 24 | 159 | 162 | 157 |
Slice thickness (mm)/gap (mm) | 2/0 | 2/0 | 4/0 | 3/0 | 4/0 |
Number of slices | 51 | 5 | 1 | 3 | 3 |
Number of excitations | 1 | 1 | 1 | 2 | 3 |
Field of view (mm) | 350 × 280 | 350 × 280 | 350 × 280 | 350 × 280 | 350 × 280 |
Base resolution (pixel) | 576 | 512 | 384 | 384 | 384 |
Phase resolution (%) | 75 | 75 | 75 | 75 | 75 |
Receiver bandwidth (Hz) | 300 | 490 | 480 | 305 | 205 |
Acquisition time | 4 min 20 s | 14 s | 2 s | 12 s | 23 s |
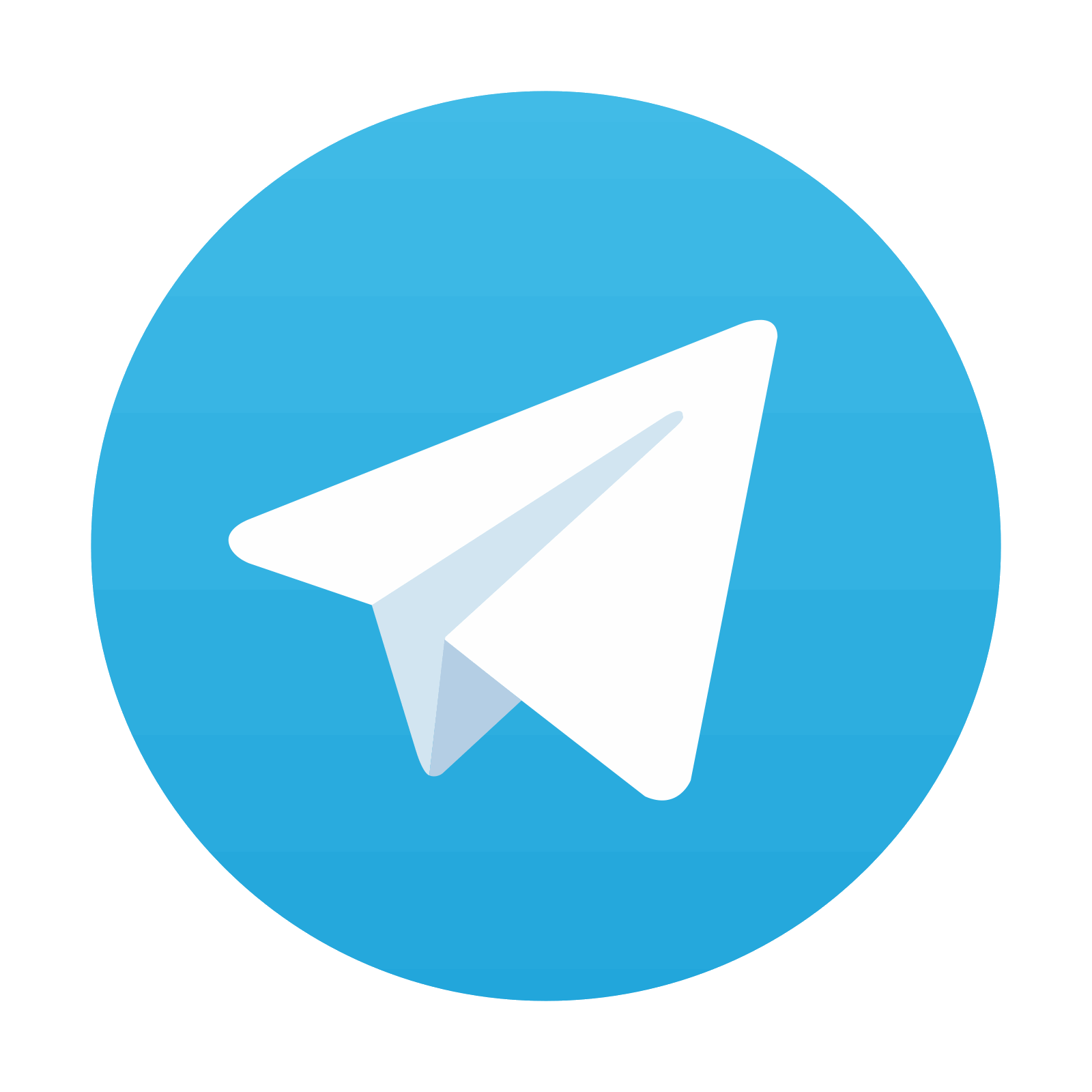
Stay updated, free articles. Join our Telegram channel
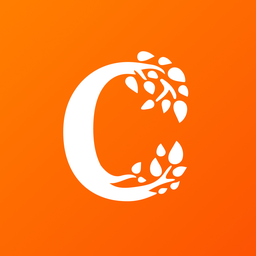
Full access? Get Clinical Tree
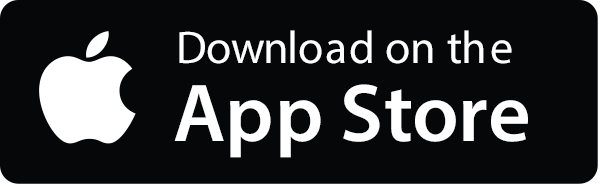
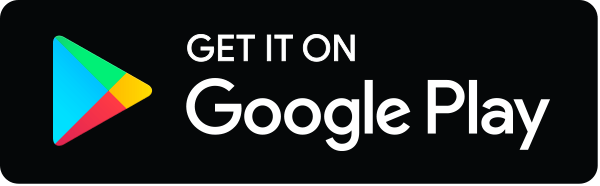
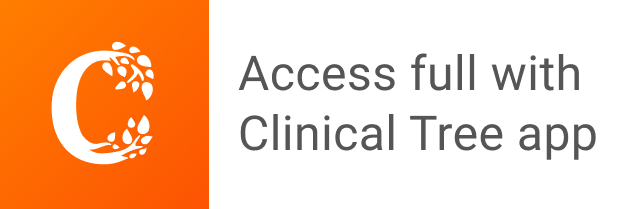