In the past decade, numerous advances in the understanding of brain tumor physiology, tumor imaging, and tumor therapy have been attained. In some cases, these advances have resulted from refinements of pre-existing technologies (eg, improvements of contrast-enhanced magnetic resonance imaging). In other instances, advances have resulted from development of novel technologies. The development of nanomedicine (ie, applications of nanotechnology to the field of medicine) is an example of the latter. In this review, the authors explain the principles that underlay nanoparticle design and function as well as the means by which nanoparticles can be used for imaging and therapy of brain tumors.
Nanoparticles are colloidal particles in which one dimension of the particle is in the range of 10 to 100 nm. Nanoparticles have become a subject for current biomedical investigation because of their unique properties, which include strong optical properties for imaging, ability to be targeted against various molecular sites, and capacity to deliver a cargo of therapeutic drugs. Although these properties have been explored in several areas of molecular and cellular imaging, applications in the neurosciences have been relatively underexplored. However, several novel imaging approaches and therapeutic advances for brain tumors are possible using nanotechnology. This article details types of nanoparticles and the means by which they may be used for imaging and therapy of brain tumors ( Table 1 ).
Particle Type | Composition | Shape | Size | Surface Coatings | Properties | Function | References |
---|---|---|---|---|---|---|---|
Liposome | Aqueous vesicle with lipid-bilayer | spherical | 100 nm–5 μm | Polymeric | Aqueous vesicular center and surface, hydrophobic bilayer, self-assembly | Delivery of therapeutic payload or imaging agent | |
Micelle | self-assembled amphiphilic lipid/polymer | spherical | 20 nm–200 nm | Hydrophilic component of amphiphile | Hydrophobic core, hydrophilic surface, self-assembly | Delivery of therapeutic payload or imaging agent | |
Iron Oxide | Fe 2 O 3 | Wide array, including sphere, rod, multifaceted | 2–60 nm core, 10–100 nm after surface | Carboxylate, silicate, polymer | Paramagnetic metal, thermally active | Magnetic resonance imaging and therapy | |
Gold | Au(0), SERS reporter | Wide array, including sphere, rod, multifaceted | 2 nm–250 nm | Carboxylate, silica, synthetic polymer, protein | Strong absorbance, plasmon resonance thermally active | Spectroscopy, imaging, therapy | |
Quantum Dots | Cd, Pd, Mg, Zn, S, Se, Te, In, P | Wide array including sphere, rod, multifaceted | 2–19 nm core; 5–40 nm after coating | Small molecule, silica, polymeric ligand, amphiphilic polymer | Bright, stable, tunable fluorescence | Imaging, investigational tool |
Nanoparticles
Liposomes and Micelles
Liposomes are formed from concentric phospholipid bilayers in aqueous conditions that yield vesicular nanoparticles. The resulting structures have a hydrophilic, aqueous core surrounded by a hydrophobic lipid bilayer, very similar to living cells. Liposomes can vary in size from 100 nm (ie, the upper limit of the nanoscale) to 5 μm, depending on the number of lipid bilayers present. In the aqueous core, hydrophilic therapeutic drugs or imaging agents can be entrapped during the self-assembly process; similarly, hydrophobic substances such as drugs or imaging agents can be incorporated into the hydrophobic shell. Such incorporation of substances is highly beneficial. For instance, incorporation of drugs maximizes drug stability, promotes unimpeded transport to the intended target, and allows for controlled and extended drug release properties. Targeting ligands can be placed on the surface of liposomes, which increases their capacity for delivery to specific tissues (eg, tumor environments). Because of their ease of preparation and biocompatibility, liposomes have found wide application for therapeutic agent or imaging agent delivery ( Fig. 1 ). In fact several US Food and Drug Administration (FDA)-approved liposome-based therapeutic agents are available on the market, including doxorubicin formulations for the treatment of Kaposi sarcoma, ovarian cancer, and multiple myeloma. Other therapeutic applications of liposomes are in various stages of clinical trials.
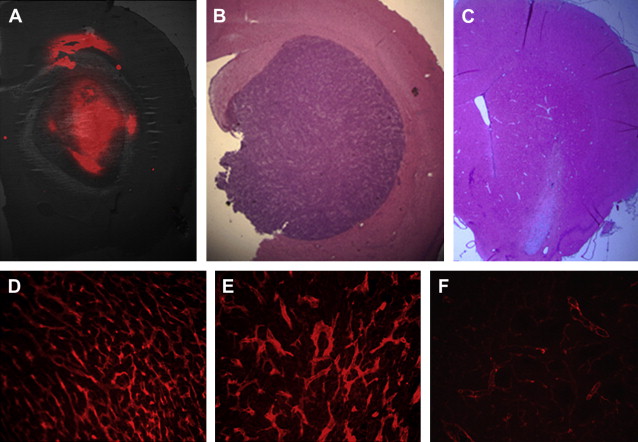
Micelles, like liposomes, are formed from the self-assembly in aqueous media of lipids or polymers that are amphiphilic (ie, have both hydrophobic and hydrophilic segments). Unlike liposomes, the amphiphilic molecules self-assemble into a structure having a hydrophobic core with a hydrophilic surface. Thus, in addition to size, the major difference between micelles and liposomes is that entrapment of a relatively hydrophobic drug occurs in the core of the nanoparticle. The major attribute of micelles is that they can make soluble therapeutic agents that are normally insoluble in aqueous or biologic environments (see Table 1 ). The hydrophilic shell provides a protective layer that allows more of the drug to be delivered to the target tissue than the administration of free drug itself. Another attribute of micelle nanoparticles is that the chemical constituents used to make them can be engineered to respond to different environments, or be sensitive to stimuli, thus controlling drug release at the desired target site.
Iron Oxide Nanoparticles
Iron oxide (IO) nanoparticles are increasingly being used for a number of imaging and therapeutic applications. These particles can be synthesized by various methods. Most commonly, they are formed by coprecipitation involving reactions with amphoteric surfactants to limit polydispersity (ie, limit the range of size, shape, and mass characteristics), which renders the nanoparticles hydrophobic. Surface modifications are needed to prevent aggregation in aqueous media. The surface atoms of IO act as Lewis acids that can be stabilized by small molecules with multiple coordinating groups, such as citric acid. Inorganic materials (eg, silica) also are used to stabilize IO nanoparticles and provide a means to covalently attach surface ligands for targeting. Polymeric coatings are yet another means of stabilizing surfaces for IO nanoparticles. Natural polymers are among the most common means of stabilizing IO particles to increase blood circulation times and allow conjugation of targeting ligands; examples of such polymers include several dextran derivatives, chitosan, and synthetic polymers, most notably poly(ethylene glycol).
Owing to their ferromagnetic core, IO particles have high relaxivity, which renders them suitable as a magnetic resonance contrast agents; some very small IO nanoparticles that are in the size range of 2 to 18 nm (termed ultrasmall paramagnetic IO, or USPIO) are used as magnetic resonance contrast agents. USPIO nanoparticles produce an increase in signal intensity on T1-weighted images comparable to the positive enhancement from gadolinium(III) complexes. Because of their high transverse relaxivity, however, these nanoparticles also decrease magnetic resonance signal on T2 -weighted images, thereby allowing them to be used with either T1-weighted imaging sequences or T2-weighted sequences ( Figs. 2 and 3 ). IO nanoparticles are among the most advanced nanoparticles in terms of translation into clinical use. Paramagnetic IO nanoparticles have already received approval by the FDA for some magnetic resonance imaging (MRI) studies of the bowel and abdominal viscera.
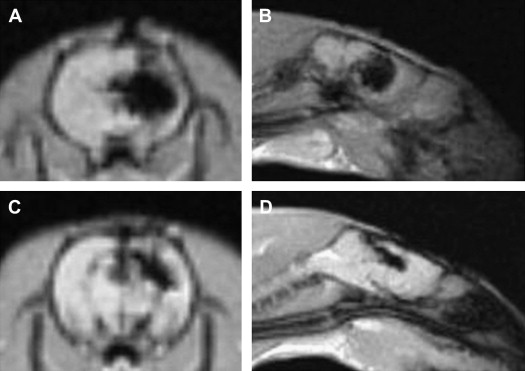
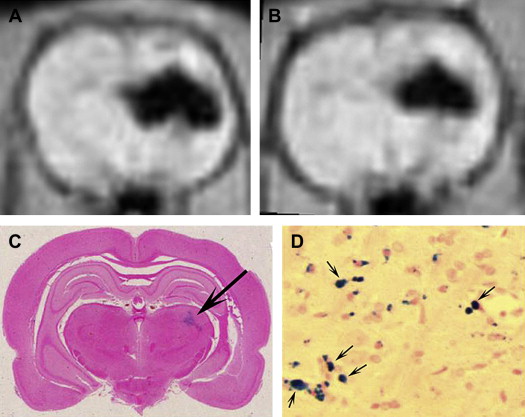
Gold Nanoparticles
Gold nanoparticles are nanoscale clusters of neutral gold atoms prepared from the reduction of gold salts in appropriate agents (eg, citric acid). This method of synthesis affords high reaction yield, good control of size, and a narrow degree of polydispersity. Because gold nanoparticles are not stable in high salt buffer, they must undergo surface modification for biomedical applications. Sulfur atoms form especially strong bonds with gold atoms. Therefore, stabilizing ligands that have a thiol group (ie, a compound that contains a functional group composed of a sulfur–hydrogen bond), such as glutathione or poly(ethylene glycol)-thiol, readily react with gold nanoparticles in aqueous conditions to increase colloidal stability. The versatile surface chemistry of gold nanoparticles allows them to be coated with various biologic targeting ligands.
Gold nanoparticles have a deep red color due to the broad surface plasmon resonance (SPR) band in the visible region (approximately 520 nm). This absorbance band is paramount to many aspects of the research performed with gold nanoparticles. The SPR effect is important, because it is the basis for many colorimetric assays using gold nanoparticles and gives information about the adsorption of materials onto the nanoparticle surface. Work by Mirkin and colleagues has used gold nanoparticles to measure biomolecule concentrations at near trace levels. Alternatively, gold nanoparticles can be used for sensitive detection assays using surface-enhanced Raman spectroscopy (SERS). SERS refers to the Raman scattering enhancement by adsorbing molecules to metal surfaces. The enhancement factor can be as large as 10 14 to 10 15 , which allows for single molecule detection.
Gold nanoparticles can be considered biologically inert, which results in very high biocompatibility. In fact, colloidal gold suspensions have been approved for human use for a number of decades to treat rheumatoid arthritis. Therefore, a wealth of research exists investigating the potential of gold nanoparticles for further in vivo development (eg, for therapeutic delivery and SERS spectroscopy). In the latter example, SERS nanoparticles are of interest because there is almost no SERS background signal with near-infrared excitation. In addition, SERS nanoparticles have a specific molecular spectra (or signature) that aids in distinguishing the nanoparticle from background fluorescence.
Finally, gold nanoparticles can be designed for absorption of light in the near-infrared portion of the light spectrum. Consequently, pulsed or continuous wave lasers have been used to selectively heat the gold nanoparticles that have been delivered to tumors for thermal ablation.
Quantum Dots
Quantum dots are semiconductor nanocrystals for which the bandgap energy (ie, the minimum energy to excite an electron above the ground state) is size-dependent; this feature endows quantum dots with unique optical and electronic properties. Quantum dots are composed of a metallic core and outer shell, which are necessary to provide the optical properties of a semiconductor. The cores commonly are comprised of cadmium sulfide, cadmium selenide, or cadmium telluride. To preserve the strong fluorescence emission of quantum dots, shells of wider bandgap energy (eg, zinc sulfide) are grown on the core; this shell provides electrical insulation and resistance to oxidation. The size, chemical composition, and interaction between core and shell can be fine-tuned to produce highly specific optical properties, such as precisely defined emission wavelengths that allow the specific type of nanoparticle to be identified. This feature allows different types of nanoparticles, each targeted against a different biomarker, to be used and discriminated from one another, which is a process termed multiplexed imaging. Quantum dots are commonly synthesized in high-temperature organic solvents that provide a surface of hydrophobic ligands. For use in biologically relevant environments, quantum dots are rendered water-soluble by several methods. Two such methods are (1) ligand exchange in a polar organic solvent followed by dialysis and (2) micelle-like encapsulation of amphiphilic lipids or polymers. For specific binding of quantum dots to different biologic targets (ie, biomarkers), quantum dots are often conjugated to targeting ligands in a final synthetic step.
The optical properties of quantum dots are characterized by broad absorbance spectra and bright, stable fluorescence emission with a narrow emission spectra. The broad absorption band allows for multiple excitation wavelengths to excite a single quantum dot and a single excitation source to excite many distinct colors of quantum dots (unlike organic dyes that have a relatively narrow absorbance band and can thus be excited solely by a single source). The narrow emission spectra of quantum dots are significant, because they allow different colors of quantum dots to be readily separated by physical filtering and computational techniques. Therefore, a single excitation source can illuminate many emission wavelengths (colors) of quantum dots. This property, combined with their brightness and enhanced photostability (ie, continued brightness after exposure to excitation sources) renders quantum dots an ideal candidate for multiplexed imaging. The increased photostability of quantum dots also makes them ideal candidates for live cell imaging; the prolonged exposure to an excitation source needed for imaging of living cells does not extinguish the bright signal emanating from quantum dots during an experiment.
Because quantum dots are composed of heavy metals, potential toxicity issues arise. If quantum dots are found to be toxic, their use in vivo will be greatly limited. Recent efforts from several groups have focused on developing biocompatible quantum dots. One approach is to minimize the length of exposure time in the body by development of quantum dots having a size small enough to guarantee rapid renal clearance; it is estimated that a size of less than 5 nm would be necessary. A second, but equally challenging, approach to increase biocompatibility is to remove many of the heavy metals while retaining semiconductor optical properties. Several groups have worked on cadmium-free or cadmium-minimized quantum dots.
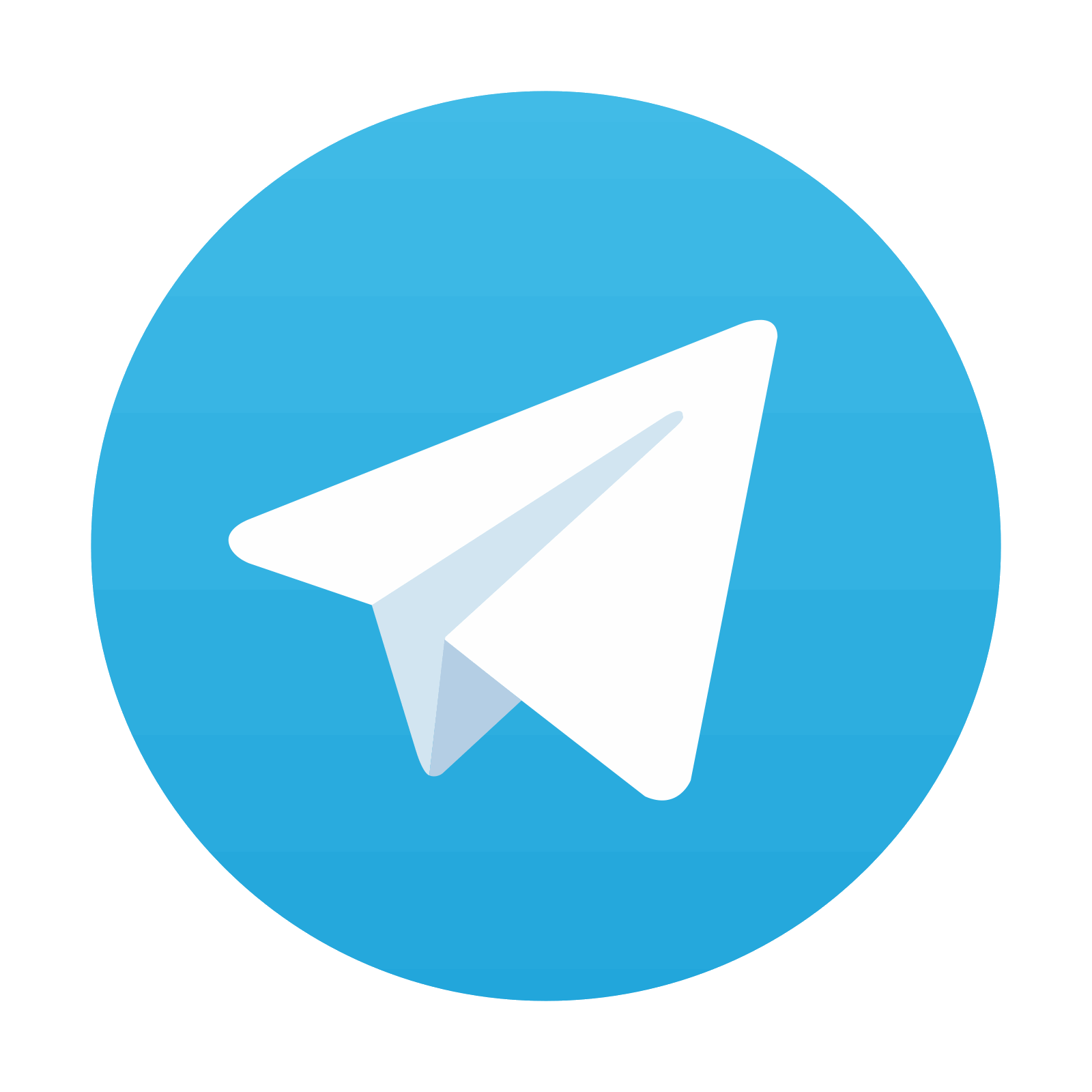
Stay updated, free articles. Join our Telegram channel
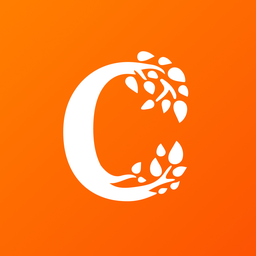
Full access? Get Clinical Tree
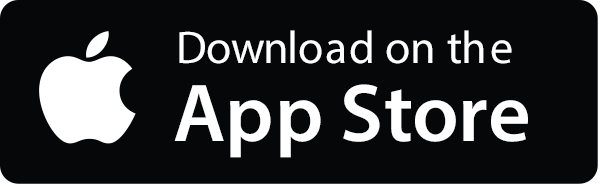
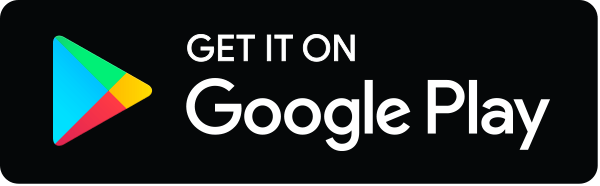