Fig. 1.1
Jablonski diagram
Both excitation and emission are observable by means of a spectrofluorometer. Within this equipment the sample is exposed to a light source emitting a constant photon flow and the excitation and emission spectra are recorded. The parameter obtained by this measure is called steady–state fluorescence intensity which is related to the fluorescence quantum yield (Φ) by the following expression:
where α is the number of photons absorbed per unit volume and I 0 is the intensity of incident light. The term steady-state refers to the condition of continuous illumination which renders a constant concentration of molecules in the excited state.

The fluorescence spectrum shows the intensity of the emitted photons correlated with the wavelength, reflecting the probability of various transitions from the lowest vibrational level S1 to different vibrational levels of S0.
Time-Resolved Spectroscopy
Fluorescence is a dynamic process developed over time after an initial electronic excitation. It decays as a function of time typically in the sub-nanosecond–nanosecond time range. Over this short period of time molecules could move, rotate, collide and participate in different reactions. The study of the dynamic of the fluorescence emission is a valuable tool to understand the mechanism of all these events. Moreover, changes in the duration of the fluorescence decay could be used to develop fluorescent sensors and markers, which could enhance the resolution and sensitivity of the conventional intensity-based fluorescence techniques.
Once a fluorophore molecule is excited it usually emits light independently, so the changes in the fluorescence intensity as a function of time are essentially concentration-independent. Moreover, the fluorescence decay is an intrinsic property of the dye, its interactions, dynamics and participation in reactions. Hence, in a simple model of identical independent fluorescence emitters, the fluorescence decay is a single exponential function of time:
where F 0 is the initial emission intensity at time t = 0. The constant τ F is the excited-state (fluorescence) lifetime. Additionally, in case of a non-exponential fluorescence decay, it is often possible to describe it as a multiexponential decay and characterize the system through an averaged life-time <τ F >.
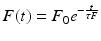
The determination of fluorescence lifetimes of samples is usually performed by two time-resolved techniques: pulsed fluorometry and phase-modulation fluorometry. The first approach uses a short exciting pulse of light and the sample response is recorded as a function of time, convoluted by the instrument response. A deconvolution routine is applied in order to recover the fluorescence lifetime parameters of the sample.
In phase-modulation fluorometry, the sample is continuously excited by a high-frequency sinusoidally modulated radiation. The fluorescence response is also sinusoidally modulated at the same frequency but phase shifted and partially demodulated with respect to the excitation (Fig. 1.2). The phase shift (ϕ) and the modulation ratio M characterize the harmonic response of the system at a given frequency (ω), and the fluorescence lifetime could be calculated from both parameters.
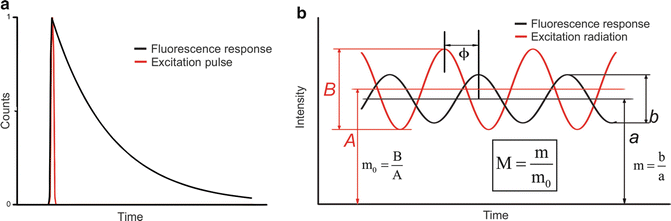
These two estimations yield the same lifetime result if the decay is truly monoexponential (i.e. a single fluorophore with amonoexponential decay). In most cases of interest, there will be multiple species with possible non-exponential decays. In such a case, these single-frequency lifetime estimations are a function of the lifetimes of the present species and the resolution of the complex system requires measurements at different modulation frequencies.
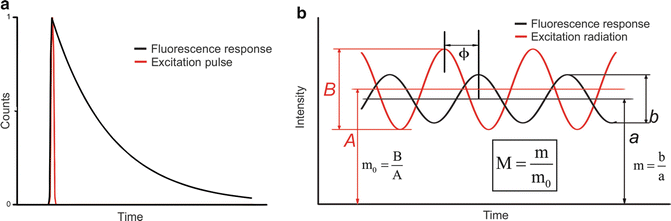
Fig. 1.2
Principles of (a) pulsed fluorometry and (b) time-resolved fluorometry
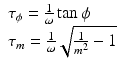
Relevant Biological Photophysical Processes
Over the last years, fluorescent molecules have been widely used as biomolecular labels, enzyme substrates, environmental indicators and cellular stains, constituting an indispensable tool in chemistry, physics, biology and medicinal sciences [5]. Owing to their high sensitivity, the detection of single fluorescent molecules and investigation of the interaction of these molecules with their local environment, the visualization of biochemical or biological process, have all became routinely possible through the use of appropriate instrumentation, such as near-field microscopy or confocal techniques. Moreover, the vast range of different types of fluorophores, the large variation in the photophysics and the chemical modifications available gives to fluorescent probe techniques an almost unlimited scope not just for in vitro and in vivo labeling of tissues, cells and intracellular structures of interest, but also for the detection of specific molecules and the investigation of intermolecular interactions on a molecular scale.
The fluorescence-based sensing is founded in the intermolecular interactions of dyes, both in fundamental and excited states, which change the optical properties of the fluorophores. These variations constitute the sensing signal of the fluorescent probes, which are reflected in changes of quantum yields, fluorescence lifetimes, and absorption and/or emission spectra of the fluorophore. Two of the most used processes in the designing of fluorescent sensors with biological relevance are Photoinduced Electron Transfer (PET) and Förster Resonance Energy Transfer (FRET).
Photoinduced electron transfer is a process frequently involved in many photochemical reactions and often responsible for fluorescence quenching. Moreover, PET processes play important roles during photosynthesis and in solar panels (Fig. 1.3).
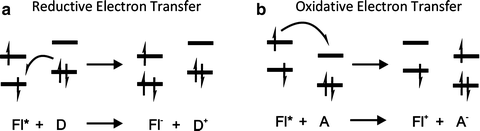
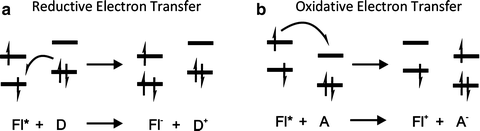
Fig. 1.3
Reductive (a) and oxidative (b) photoinduced electron transfer (PET)
When the fluorophore is excited by the absorption of a quantum of light (Fl*), the molecules gain energy, which allows its participation in different reactions. Therefore, the excited fluorophore molecules could be considered as redox sites that can donate or capture electrons from other species, being either oxidant or reductant. Photoinduced Electron Transfer is the process by which an electron is transferred from one excited site to specie. Two important requirements for this reaction are the closeness in space of the two sites and the matching of their redox potentials. PET can be facilitated by covalent bonding between donor and acceptor via a short spacer. Usually in PET systems just one of the states (reactant or product) is fluorogenic, consequently the reactions lead to a quenching or enhancement of the fluorescence emission of the sample.
Förster Resonance Energy Transfer (FRET) is a process that involves the non-radiative transfer of energy from a photoexcited specie, which is the energy donor, to another specie performing as the energy acceptor. FRET efficiency depends strongly upon the donor–acceptor distance and the spectral overlap integral between the emission spectrum of the donor and the absorption spectrum of the acceptor.
Fluorescence Microscopy Techniques
A fluorescence microscope is a type of optical microscope that uses the fluorescence properties of the samples instead of – or in addition to – the absorption and reflection. For this purpose, a given fluorescent or fluorescently labeled sample is illuminated with a wavelength-specific excitation radiation and the emitted light is separated using a defined array of filters and dichroic mirrors, known as “cube.” The “cube” shown in Fig. 1.4 is composed of an excitation filter, that allows only the right wavelength light to pass throw; a dichroic mirror displayed at a 45° angle that reflect the excitation light but permits the emitted light to pass; and an emission filter that allows to pass the desired wavelength light. Cubes are available or could be set up to match the spectral excitation and emission of the fluorophore used in the sample (Fig. 1.4).
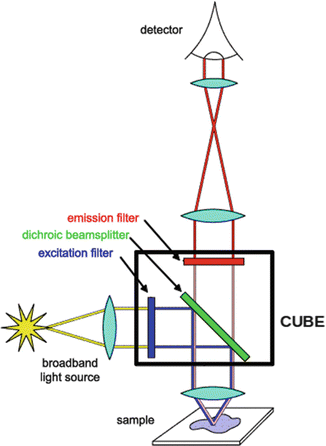
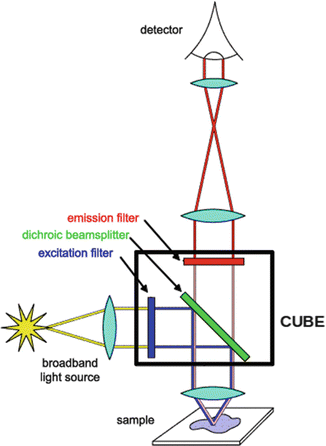
Fig. 1.4
Schematic representation of the working principle of a fluorescence microscopy showing the cube containing dichroic mirror, excitation, and emission filter
Epifluorescence Microscopes
These are the simplest type and mostly used florescence microscopes, in which the excitation and emission light go through the same light path. The light sources used in these microscopes are usually xenon arc lamps, mercury-vapor lamps or more recently high power LEDs. These sources have discreet emission lines that allow to, with the right cube, excite the sample with monochromatic or near-monochromatic light.
Confocal Microscopes
These are microscopes that achieve by a variety of methods the optical sectioning of the sample to get a better resolution of the image by eliminating out of focus emission. Confocality is reached by point illumination and detection using a pair of optically conjugate pinholes. For this purpose, the most widely used method is the confocal laser scanning microscope that combines a laser to excite point by point the sample and a fixed pinhole and detector. The scanning and descanning are achieved by using a set of computer-controlled mirrors and the image is reconstructed by a special software. Alternatives to the laser scanning microscope are the spinning disk microscope and the Programmable Array Microscope (PAM). The first one uses a set of moving pinholes on a spinning disk to scan the sample and the second one an array of micro-mirrors to generate a computer-controlled illumination pattern. Both microscopes usually use a CCD camera to acquired image and imaging frame rates are faster than the laser scanning systems (Fig. 1.5).
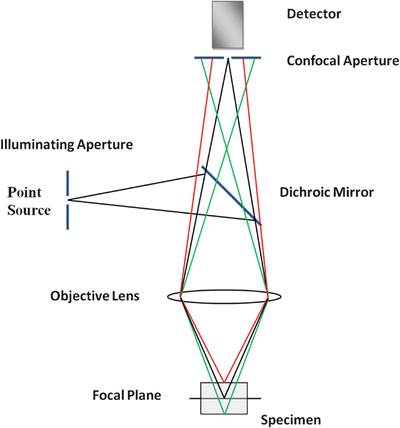
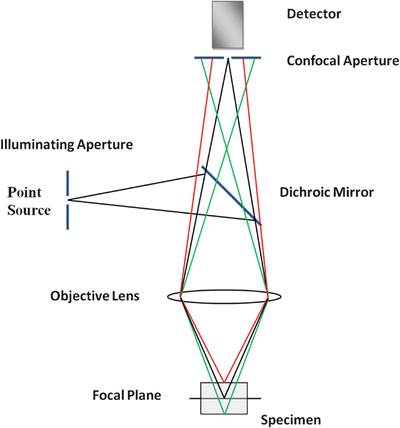
Fig. 1.5
Schematic representation of a confocal microscope. The incident light illuminates a single point on the focal plane of a specimen (black), the fluorescent light emitted passes through the dichroic mirror and the conjugated pinhole allows only the in-focus light from the region of interest on the sample to pass through to the detector. The light rays colored red and green in the figure represent the fluorescent emission from different focal planes
Two-Photon Excitation Microscopy
This microscopy technique uses a simple concept based on the fact that a fluorophore can be excited by two photons of carrying half of the energy needed. These lower energy photons are provided by infrared light (typically between 700 and 1,000 nm), allowing tissue imaging by an increased penetration depth since most of the tissues are transparent to this light. This excitation also minimized tissue damage and provides low background signal. Therefore, as appose to the conventional fluorescence techniques, the excited molecules emit fluorescence at a lower wavelength (higher energy) than the excitatory photons.
Since the fluorophore has to be excited simultaneously by two photons, this technique requires a high flux of excitation light that is provided by a laser source focused in one plane. This fact minimized out of focus fluorescence and provides a lower spread of the point-spread-function than single-photon excitation, resulting in an improved resolution along the z axis.
Molecular Fluorescent Probes for Imaging in the NIR Region
Imaging biological systems by fluorescence techniques became the most expanded tool to evidence and understand how a variety of biological events take place within a cell, tissue, or whole organism. Fluorescence determinations are especially sensitive and non-invasive and allow the observation of processes in real time with spatial resolution. Compared to absorbance measurements, the detection of fluorescence emission can be achieved at much lower probe concentrations and among imaging techniques fluorescence instrumentation is less expensive, simple and adaptable.
Some biomolecules, like proteins, contains intrinsic fluorophores like the amino acid tryptophan, but they are mainly not useful especially because of poor image contrast. Extrinsic molecular fluorophores ranging from visible to near infrared emission are widely preferred due to the versatility available when it comes to optical properties (absorption/emission, quantum yields, photostability) and the possibility to get them specifically attached to the target biomolecule. Compared to other extrinsic fluorophores like genetically encodable fluorescent proteins or fluorescent nanoparticles (Quantum dots), organic molecular probes have the advantage of being small in size, which leads to negligible perturbation of the structure or function of the labeled biomolecule.
Fluorescent probes are used for many different imaging applications using conventional fluorescence techniques [8] and more recently by advanced microscopic methodologies like single-molecule [9] or superresolution [10] techniques.
Ideally, a molecular fluorescent reporter should have high brightness that allows spatial and temporal resolution, high photostability in order to have a good observation window, and related to biological applications it should be non-toxic. Besides these basic requirements and foreseeing the application in image-guided surgery, the fact of being near infrared (NIR) emitters and with appropriate derivatization for specific labeling are high rated characteristics.
NIR fluorescent probes emit photons in the region between 650 and 900 nm. The main advantage of working in this range is that tissue auto-fluorescence in living systems are avoided (e.g. emission of elastin, collagen, hemoglobin). Therefore, a higher signal-to-noise ratio is accomplished leading to a better observation at the molecular level. Additionally, reduced light scattering and enhanced tissue penetration are achieved.
NIR emitting fluorophores have been mainly used in bioimaging applications such as pH fluctuations, ion sensing and multicolor imaging [11] in living cells with very successful results. The objective of translating these studies from the molecular biology to the medical field is in continuous growing since the last 5 years. Research made by different groups focus on the design of the chemical structure and optical properties of a fluorophore to meet the requirements for a probe to be successful as an imaging agent in surgery. Regarding clinical use, only Indocyanine Green (ICG) and Methylene Blue (MB) have received FDA approval (Fig. 1.6).
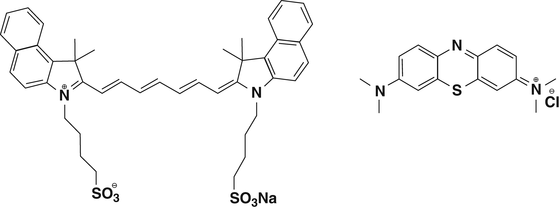
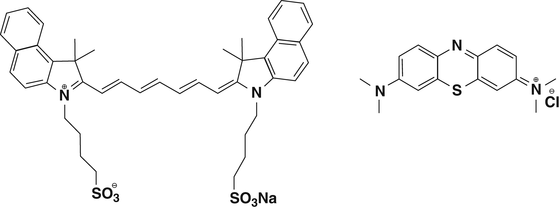
Fig. 1.6
Chemical structure of Indocyanine Green (left) and Methylene Blue (right)
MB is a phenotiazine with lower quantum yield and extinction coefficient than ICG. However, it has been used in surgery to identify ureters [12], extrahepatic bile ducts [13], among others.
ICG belongs to the heptamethine cyanines family and its use as surgical imaging agent is more expanded as will be discussed later on. The cyanine dyes are extensively studied and found application in a variety of research fields. One of the reasons of their widespread use is the chemical versatility they possess and that can be exploited to tune in a desired optical property or for further conjugation with a fragment of interest.
An important class of NIR dye are rhodamine and xanthene analogs, which have been chemically modified to shift their absorption/emission bands to the near infrared region. Although these dyes have excellent quantum efficiency they suffer from some disadvantages such as poor water solubility and short Stokes shifts. Recently, some progress has been made by Nagano et al. to overcome some of these issues [14].
There are other families of NIR dyes that are intensively used as sensors or probes for imaging cell cultures and tissues like: BODIPY analogs, Squaraine, Perylene derivatives, etc. [15]. Most of the research made on the design and synthesis of these molecules focus on the improvement of properties such as aqueous solubility, better photophysical parameters and convenient derivatization for specific labeling of biomolecules. Given the demand for new NIR probes coming from biomedical research is mandatory to study biocompatibility and pharmacokinetic of a probe intended to be applied as a diagnostic or treatment tool. Regarding imaging agents for a surgical procedure, according to Nguyen and Tsien, the probe is better to be administered topically or intravenously before operation and it should render high imaging contrast during the procedure or at the moment of tissue resection [16].
Although in its infancy, NIR imaging applied to surgical procedures will provide surgeons a real-time guidance to the target tissue for resection or to identify tissue that should be avoided. The reader is encouraged to seek up the review by Gioux et al. for further reading on the influence of key parameters of the imaging system for NIR fluorescence-guided surgery [17]. About light sources and hardware associated with these procedures, we recommend the reviews from Nguyen and Berg [16, 18].
NIR Molecular Probes for Imaging Guided Surgery
As mentioned before, ICG is actually the most widely used NIR imaging probe in intraoperative proceedings. Alander et al. have reviewed over 200 papers reporting on the use of this dye as a fluorescent probe, stressing the increase of publications related since 2009 [19]. Schaafsma et al. reported in the same year an extensive revision of the bibliography referred to the use of ICG in cancer-related surgery [20].
New successful applications of ICG were very recently published. For example, the use of this dye for assistance during a hepatic metastasectomy allows to identify superficially located colorectal liver metastases and in some patients it was possible to localize small lesions otherwise undetectable by typical procedures [21].
Here we present relevant potential imaging agents inspired by ICG which were reported the last 2 years, to illustrate how current disadvantages of ICG could be overcome following a rational chemical design.
Ebert & colleagues reported in 2011 a sugar derivative of ICG named SIDAG which foreseen in its design a superior hydrophilicity than ICG [22]. As a consequence SIDAG showed renal elimination, an acute tolerance 60-fold higher and strong tumor contrast in rat breast carcinomas.
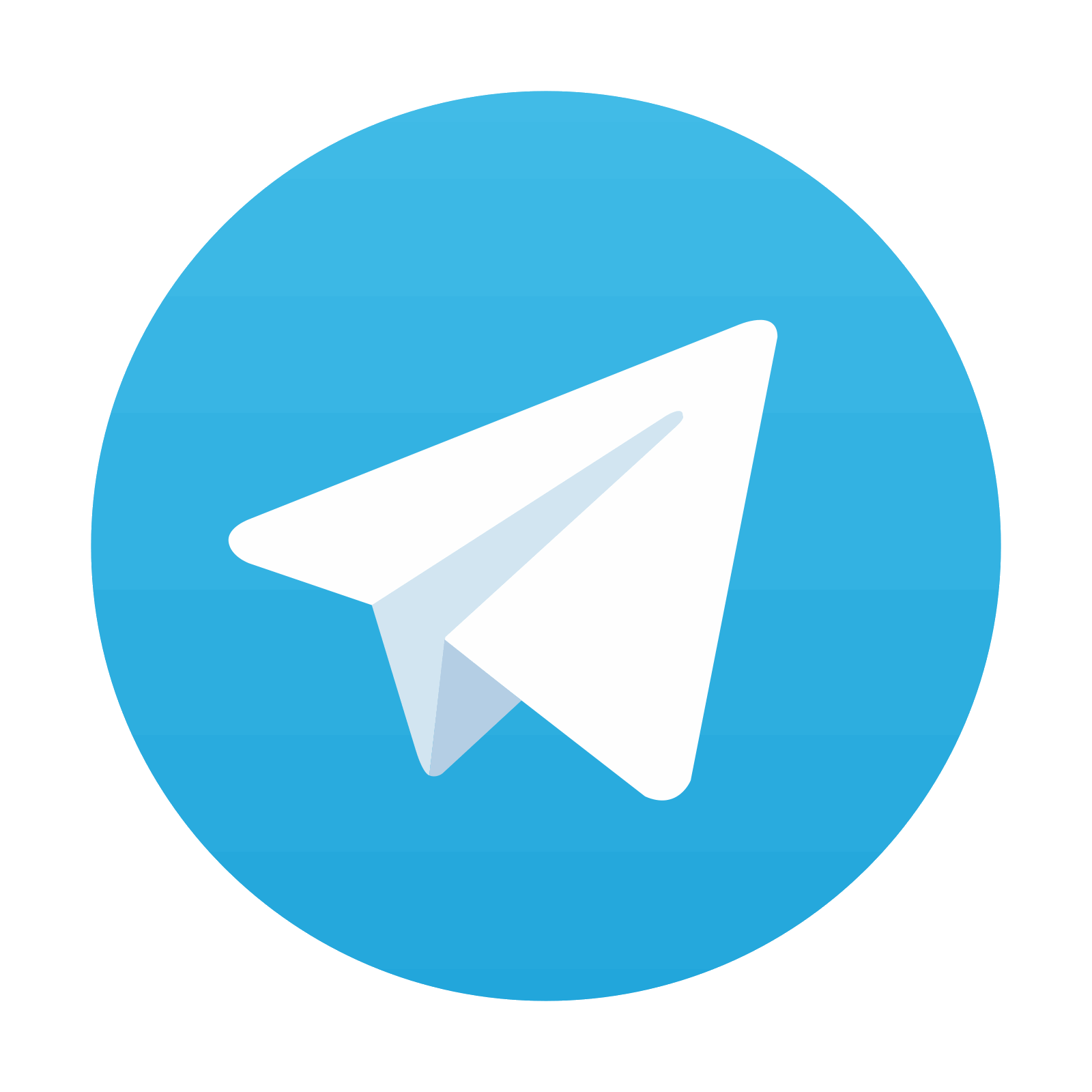
Stay updated, free articles. Join our Telegram channel
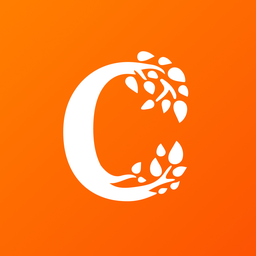
Full access? Get Clinical Tree
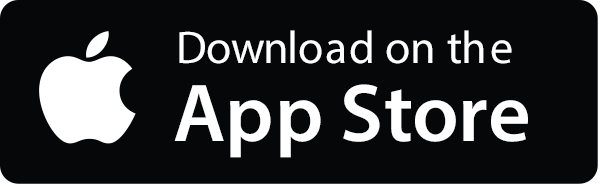
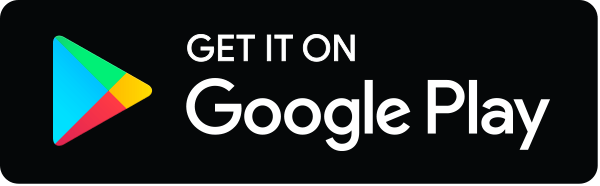