where BED is the biologically effective dose as a measure of the true biological dose delivered by a particular RT regime, n the number of treatment fractions and d the dose per fraction. To understand by intuition, the comparison of schedules consisting of different total doses or doses per fraction is also possible by converting each RT schedule into an equivalent schedule in 2Gy-fractions, which would give the same biological effect:
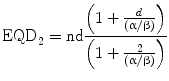
2.3 Patient Selection for HDR Brachytherapy
Based on the assumption that failure to eradicate organ-confined prostate cancer may lead to the development of metastatic disease, patients without advanced disease may benefit most from dose-escalated RT in terms of improved LC, BC, and MFS (Zelefsky et al. 2008a, b, 2011; Kupelian et al. 2008; Coen et al. 2002; Kim et al. 2012; Nguyen et al. 2013; Pahlajani et al. 2012). Accordingly, the main indication for HDR treatment is histologically proven localized prostate cancer in patients considered otherwise suitable for radical treatment (Yamada et al. 2012; Hoskin et al. 2013). Mature results exist for the application of HDR BRT as a boost modality in combination with EBRT for intermediate- and high-risk disease. In addition, evolving clinical evidence implicates its effective use as monotherapy with encouraging results reported for mainly low—and intermediate—but also selected cases of high-risk disease. However, monotherapy with curative intent for high-risk disease should still be considered investigational and more mature data are needed to confirm its role. In the clinical setting of regional lymphadenopathy with or without distant disease spread, HDR BRT may be implemented combined with EBRT in individualized treatment schemes in order to avoid increased toxicity where conventional conformal EBRT is employed with the goal of LC.
Pre-treatment investigations for HDR BRT should be no different from those for other forms of radical treatment and should follow the EAU guidelines (Hoskin et al. 2013; Heidenreich et al. 2011). All patients considered for IRT irradiation should have histological confirmation of malignancy. Staging tests for evaluation of prostatic disease burden and of any extraprostatic extension should include digital rectal examination, transrectal ultrasound (TRUS) and, if indicated, computed tomography (CT) and/or magnetic resonance imaging (MRI) and bone scintigraphy. In equivocal cases of regional lymphadenopathy, laparoscopic pelvic lymphadenectomy (Touijer et al. 2011) or choline positron emission tomography (Evangelista et al. 2013) may be considered. Even though functional outcome is predicted by the baseline urinary function (Eid et al. 2013), neither larger gland size nor previous transurethral resection of the prostate (TURP) are absolute contraindications for treatment. Transperineal implantation in lithotomy position enables the complete and safe coverage of prostate volumes appreciably greater than 60 ccm provided a sufficiently broad pelvic inlet and freedom from lower urinary tract symptoms (LUTS) requiring treatment (Yamada et al. 2012; Le et al. 2013; White et al. 2013; Monroe et al. 2008; Rogers et al. 2012). In addition, temporary IRT implantation with HDR irradiation is safely feasible at >3 months after TURP given freedom from persistent LUTS and provided a sufficient amount of residual gland volume for image-based three-dimensional (3D) treatment planning including precise organs at risk identification (Luo et al. 2009, 2013; Peddada et al. 2007; Ishiyama et al. 2012). However, limited resection-TURP may also be part of an interdisciplinary patient management prior to planned HDR BRT when a prominent median lobe is encountered during staging. Selection criteria for HDR BRT combined with EBRT or as monotherapy are shown in Table 1. Unlike for permanent LDR implants, in temporary HDR BRT the IRT catheters can be placed next to extracapsular lesions or the seminal vesicles also into the bladder pouch, if necessary. Therefore, the indication for HDR BRT is by various groups extended to even T4 tumors (Kotecha et al. 2013; Yoshioka et al. 2011, 2013; Sakamoto et al. 2011). Nevertheless, in cases of previous pelvic EBRT or surgery resulting in anatomical changes of the organs of the small pelvis, a very careful evaluation of the potential risks and benefits should be performed.
Table 1
Patient selection criteria for image-guided high-dose-rate brachytherapy in the treatment of clinically localized or locally-advanced prostate cancer
Inclusion criteria |
Stages T1–T3ba |
Any GS |
Any PSA level |
Exclusion criteria |
TURP within 3 months |
Urinary flow rate <10 ml/s |
IPSS > 20 |
Pubic arch interferenceb |
Lithotomy position not possiblec |
Anesthesia not possible |
Rectal fistula |
3 Brachytherapy Techniques
Interstitial catheter insertion is usually performed under spinal or general anesthesia with various catheter placement patterns being described. Extensive experience exists for the technique of real-time TRUS-guided implantation (Zamboglou et al. 2012; Demanes et al. 2009, 2011; Hoskin et al. 2012; Martinez et al. 2002; Lee 2009; Corner et al. 2008; Ghilezan et al. 2011), however, MRI-based techniques have also been implemented in clinical practice (Ménard et al. 2004; Lakosi et al. 2011).
In general, implant dosimetry is based on 3D image data sets for definition and delineation of the target volume (prostate gland = planning target volume, PTV) and the organs at risk (OAR’s, i.e., intraprostatic urethra, anterior rectal wall, and urinary bladder). The clinical workflow includes the creation of virtual volumes prior to implantation for inverse treatment pre-planning and volume studies after catheter insertion for treatment planning prior to irradiation (Mavroidis et al. 2010; Karabis et al. 2005). Treatment planning after TRUS-guided implantation is most commonly performed using either CT- (Martinez et al. 2002; Demanes et al. 2005; Galalae et al. 2002; Hoskin et al. 2007) or TRUS-imaging (Zamboglou et al. 2012; Demanes et al. 2011; Hoskin et al. 2012; Ghilezan et al. 2011). For CT-based planning, the image data set should be contiguous with no more than 3 mm slice thickness in the axial plane. Imaging should extend the prostate in the craniocaudal extension and should include sufficient normal anatomy for meaningful normal tissue dosimetry (Yamada et al. 2012). When more than one implantation is performed, it is not uncommon for the prostate to have varying volumes between the procedures. This has to be taken into account by planning each BRT fraction separately. In contrary to the totally TRUS-based clinical workflow with intraoperative real-time treatment planning, CT-based planning bears an additional risk for catheter re-arrangement and target volume changes due to transferring of the patient to a CT-scanner, which constitutes a deviation of the patient setup (Whitaker et al. 2011; Holly et al. 2011; Seppenwoolde et al. 2008). From this perspective, the totally TRUS-based technique illustrates the standard of care in prostate BRT ensuring improved 3D implant stability (Milickovic et al. 2011).
Representatively described, transperineal implantation is performed under TRUS-guidance using a continuous probe movement technique and a perineal template to aid catheter placement (Martin et al. 2004). For pre-planning, transversal ultrasound images of the prostate, urethra, and anterior rectal wall are acquired in real-time and 3D volumes are reconstructed based on 1.0 mm image distance. The PTV is defined as the entire prostate gland without margins. Contour definition for the rectum extends 10 mm cranially from the prostatic base and 10 mm caudally from the prostatic apex. Urethra contouring encounters the intraprostatic urethra marked by the insertion of a radiopaque three-way Foley catheter and extends by 5 mm caudally to include the apical membranous urethra. The urinary bladder is contoured based on the TRUS-based visible volume. Based on the acquired 3D anatomy, the appropriate virtual needle positions are generated, the needle source dwell positions located within the PTV are activated, and the radioactive source dwell times are calculated using an intraoperative treatment planning system (Fig. 1). Dose volume histograms (DVH’s) for the PTV and OAR’s are calculated for evaluation of the anatomy-based dose optimisation. If the pre-planning dosimetry parameters fulfill the dosimetric protocol, TRUS-guided implantation of catheters is performed at the previously determined positions (Fig. 2). After completion of implantation, a final 3D TRUS data set is acquired for intraoperative real-time treatment planning. Planning target volume and OAR’s contouring is checked and updated according to the new image set. Real needle positions are reconstructed and dwell positions located within the PTV are activated ensuring the 3D dose distribution fulfills the dosimetric protocol (Fig. 3). The prescribed dose is the intended minimum dose delivered to the PTV surface.
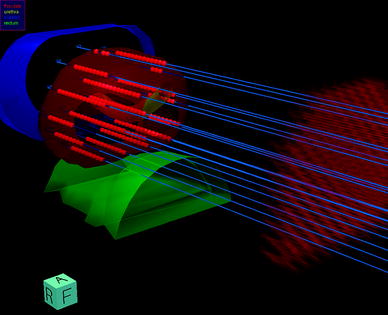
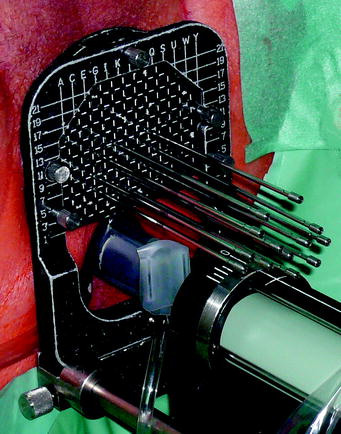
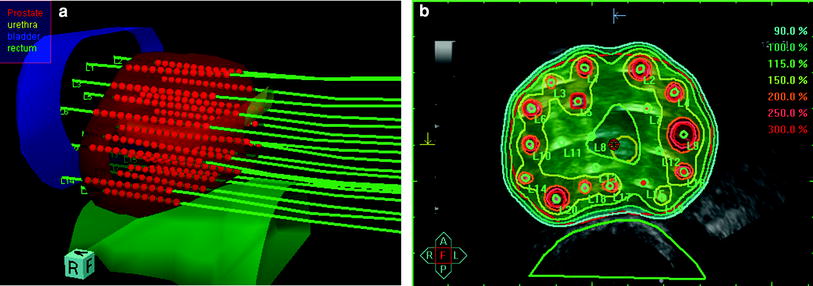
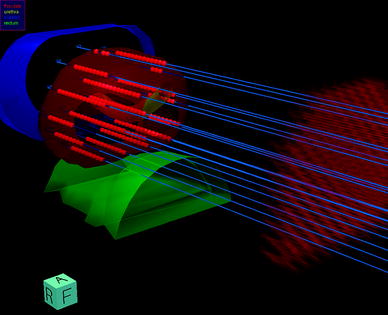
Fig. 1
Three-dimensional reconstruction of the prostate, urethra, rectum, and bladder with ideal template needle trajectories for TRUS-guided implantation as calculated for pre-planning by the real-time treatment planning system SWIFT/Oncentra Prostate (Nucletron B.V., Veenendaal, The Netherlands). The virtual perineal template is displayed on the right side
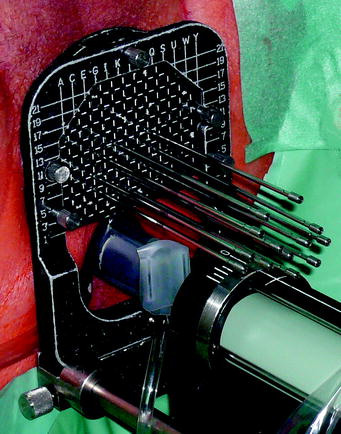
Fig. 2
High-resolution template with implanted interstitial steel needles (200 mm length, 1.9 mm diameter). The transducer probe for TRUS-guidance is attached to a floor-mounted stepping unit and inserted into the rectum. A thin intestinal tube for the discharge of intestinal gases is inserted laterally to the probe and is partly visible behind the lower right angle of the template
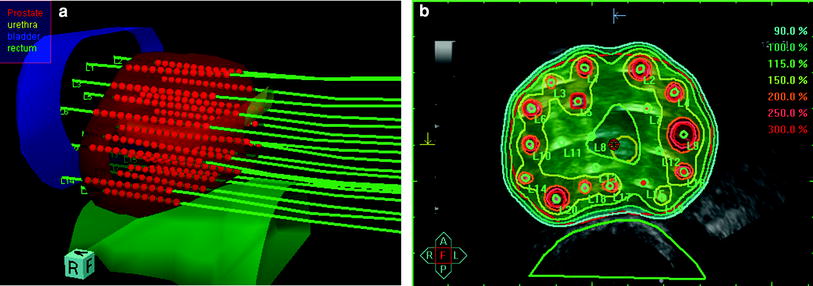
Fig. 3
Intraoperative real-time TRUS-based treatment planning. a Three-dimensional reconstruction of the prostate, organs at risk (i.e., rectum, urethra, bladder), in situ needles and the intraprostatic source dwell positions as calculated using the real-time treatment planning system SWIFT/Oncentra Prostate for the final treatment plan; b Isodose distribution after anatomy-based dose optimisation. The isodose color code convention is: dark red 300 % {isodose = 28.5 Gy}; orange 200 % {isodose = 19.0 Gy}; yellow 150 % {isodose = 14.25 Gy}; turquoise 100 % {isodose = 9.5 Gy}. The 100 % isodose was set to encompass the red delineated prostate capsule
Given the extreme heterogeneity in clinically implemented dose-fractionation schedules, it is difficult to establish absolute dose guidelines for total physical prescription doses and normal tissue dose constraints. For comparison, Table 2 lists dose-fractionation schedules and normal tissue dose constraints used by various centers. For example, the William Beaumont Hospital monotherapy protocol consists of one IRT implant that delivers four fractions of 9.5 Gy in 2 days under spinal anesthesia with an interfractional interval of at least 6 h (Demanes et al. 2011). The current Offenbach Hospital monotherapy protocol consists of three implants each delivering one fraction of 11.5 Gy under spinal anesthesia with all implants separated by 21 days (Zamboglou et al. 2012). The Mount Vernon Cancer Center combined treatment protocol consists of 35.75 Gy EBRT delivered in 13 fractions followed within 6 days by an HDR boost. Interstitial implantation is performed under general anesthesia with one implant delivering a total dose of 17 Gy in two fractions within 24 h (Hoskin et al. 2007). At the Catalan Institute of Oncology, combined treatment is delivered with 60 Gy conventionally-fractionated EBRT followed within 21 days by a single-fraction HDR boost of 9 Gy under spinal anesthesia (Pistis et al. 2010).
Table 2
Total physical prescription doses and normal tissue dose constraints (as percent of prescribed high-dose-rate brachytherapy reference dose or absolute dose value) of various brachytherapy schemes
Treatment protocol | PTV | Rectum | Bladder | Urethra | D90 (%) | V100 (%) | V150 (%) |
---|---|---|---|---|---|---|---|
Demanes et al. (2011)a | |||||||
CET | 7.0 Gy × 6 (42 Gy) | D0.1 ccm ≤ 80 % | D0.1 ccm ≤ 80 % | D0.1 ccm ≤ 110 % | >100 | >97 | – |
WBH | 9.5 Gy × 4 (38 Gy) | D0.1 ccm ≤ 75 % | D0.1 ccm ≤ 80 % | D0.1 ccm ≤ 120 % | >100 | >96 | – |
Zamboglou et al. (2012)a | 9.5 Gy × 4 (38 Gy) | D0.1 ccm ≤ 80 % | D0.1 ccm ≤ 80 % | D0.1 ccm ≤ 120 % | ≥100 | ≥90 | ≤35 |
11.5 Gy × 3 (34.5 Gy) | D0.1 ccm ≤ 80 % | D0.1 ccm ≤ 80 % | D0.1 ccm ≤ 120 % | ≥100 | ≥90 | ≤35 | |
Prada et al. (2012)a | 19 Gy × 1 (19 Gy) | D 0.1 ccm ≤ 75 % | – | D 0.1 ccm ≤ 110 % | – | – | – |
Hoskin et al. (2007)b | 37.7 Gy EBRT + 2 × 8.5 Gy (17 Gy) BRT | D2.0 ccm < 6.7 Gy | – | D10 < 10 Gy | – | – | – |
Kotecha et al. (2013)b | 45–50.4 Gy EBRT + 3 × (5.5–7.5 Gy) BRT | D0.1 ccm ≤ 100 % | – | D0.1 ccm ≤ 120 % | – | ≥100 | – |
Pistis et al. (2010)b | 60 Gy EBRT + 1 × 9 Gy BRT | D2.0 ccm ≤ 75 % | – | D2 % < 120 % | ≥105 | ≥98 | ≤50 |
In MRI-based techniques, catheter placement is performed transperineally and maximum insertion depth, direction, and positional control of the implanted catheters are estimated by interactive MR-scanning (Ménard et al. 2004; Lakosi et al. 2011). Number, geometrical alignment, and distance between the catheters depend on the size/shape of the prostate and the alignment of the intraprostatic urethra. Total physical prescription doses and dose restrictions for OAR’s are in accordance with the protocols listed in Table 3. Irrespective of the imaging modality for interventional guidance or treatment planning, IRT HDR irradiation is performed using a remote afterloading system. Iridium 192 is the most commonly used isotope with an average energy of 380 keV, a half-life of 73.8 days and a half value layer of 2.5 mm of lead (Nag et al. 2003).
Table 3
Literature results of high-dose-rate brachytherapy as boost modality to external beam radiotherapy for localized prostate cancer
Treatment scheme | ||||||
---|---|---|---|---|---|---|
Study | n | Total EBRT dose (Gy/fx) | Total HDR dose (Gy/fx) | Total BED/EQD2 (Gy) | Follow-up (y) | Biochemical controla |
Galalae et al. (2002) | 144 | 40/20 | 18/2 | 219/94 | Median 8.2 | 74 %/69 % all risk groups at 5 years/8 years |
Pistis et al. (2010) | 114 | 60/20 | 9/1 | 203/87 | Mean 2.7 | 97.4 % IR and HR at 4 years |
Kotecha et al. (2013) | 229 | 45–50.4/25–28 | 16.5–22.5/3 | 171–226/74–97 | Median 5.1 | 95 % LR at 7 years, 90 % IR at 7 years, 57% HR at 7 years (81 % HR with BED > 190 Gy) |
Martin et al. (2004) | 102 | 45/25 | 20–28/4 | 191–251/82–108 | Median 2.6 | 100 % LR/IR at 3 years, 79 % HR at 3 years |
Prada et al. (2012) | 252 | 46/23 | 21–23/2 | 292–366/109–137 | Median 6.1 | 84 %/78 % HR at 5 years/10 years |
Åström et al. (2005) | 214 | 50/25 | 20/2 | 269/116 | Median 4 | 82 % all risk groups at 5 years |
Martinez et al. (2002) | 207 | 46/23 | 16.5–23/2–3 | 184–306/79–131 | Mean 4.4 | 52 % all risk groups for EQD2 < 93 Gy and 87 % all risk groups for EQD2 > 93 Gy at 5 years |
Noda et al. (2011) | 59 | 50/25 | 15–18/2 | 191–243/82–104 | Median 5.1 | 100 % LR at 5 years, 92 % IR at 5 years, 72 % HR at 5 years |
Hoskin et al. (2007) | 220 | 35.75/13 | 17/2 | 214/92 | Median 2.5 | Mean PSA relapse-free survival for all risk groups 4.3 years |
4 Clinical Data
4.1 HDR Boost in Combination with EBRT
In patients with organ-confined prostate cancer, radiation dose escalation has shown to improve both LC and BC (Kuban et al. 2008; Dearnaley et al. 2007; Zelefsky et al. 2008, 2011; Peeters et al. 2006; Kupelian et al. 2008; Kim et al. 2012; Nguyen et al. 2013; Pahlajani et al. 2012; Pollack et al. 2002; Zietman et al. 2010). However, the benefits of local dose escalation must be weighed against the risks of toxicity to the surrounding healthy tissues. The combination of EBRT and HDR BRT allows the safe delivery of high biologic equivalent doses to the prostate not achievable in terms of conformality even by adaptive image-guided IMRT (Kupelian and Meyer 2011).
Two randomized trials have helped to show the superiority of HDR BRT combined with EBRT over EBRT alone in the radical treatment of localized prostate cancer. In the mid-1990s, Sathya et al. (Sathya et al. 2005) assigned 104 patients to conventional EBRT of 66 Gy in 33 fractions or to 35 Gy HDR BRT to the prostate with supplemental conventional EBRT of 40 Gy in 20 fractions. At a median follow-up of 8.2 years, the authors reported biochemical failure in 17 patients of the combined arm compared with 33 patients in the EBRT alone arm (P = 0.0024). Overall survival was 94 % in the combined arm versus 92 % in the EBRT arm without statistically significant differences in acute and late toxicity. The authors reported 2.0 % Grade 3–4 genitourinary (GU) and 3.9 % Grade 3–4 gastrointestinal (GI) adverse events at 18 months for the combined arm compared to 3.8 and 1.9 %, respectively, for the EBRT arm. Almost a decade later, Hoskin et al. (2007) randomized 220 patients to receive either hypofractionated EBRT alone or hypofractionated EBRT plus HDR BRT. The EBRT group (n = 111) received 55 Gy in 20 fractions, whereas the combined treatment group (n = 109) was given 35.75 Gy EBRT in 13 fractions plus a 17-Gy HDR boost applied in two fractions within a single implant. With a median follow-up of 30 months, a significant improvement in actuarial biochemical relapse-free survival was seen in favor of the combined treatment schedule. The mean biochemical failure-free survival in the BRT arm was 5.1 versus 4.3 years in the EBRT only group (p = 0.03) with no significant difference in late bowel or bladder reactions between the two arms when considering Grade 2 and greater severity. The results of these randomized trials are in line with the oncological outcomes in large retrospective series reporting promising long-term biochemical relapse-free survival rates (Pistis et al. 2010; Kotecha et al. 2013; Prada et al. 2012). Pistis et al. (2010) treated 114 patients with intermediate- and high-risk prostate cancer using a treatment schedule consisting of mean 60 Gy conventional EBRT, followed 3 weeks later by a single-fraction 9 Gy HDR boost yielding a total combined BED1.5 of 203 Gy. The generated overall BC at 4 years was 97.4 % with no higher grade acute or late GI and GU adverse events. Kotecha et al. (2013) treated 229 patients with an HDR BRT boost followed 3 weeks later by supplemental EBRT. The boost consisted of three fractions BRT of 5.5–7.5 Gy per fraction and supplemental EBRT of conventionally fractionated IMRT delivering 45.0–50.4 Gy. The generated BED1.5 levels ranged from 171 to 226 Gy with a median value of 191.5 Gy. The 7-year biochemical relapse-free survival rates were 95, 90, and 57 % for low-, intermediate-, and high-risk patients, respectively, with a 7-year BC of 81 % among high-risk patients with BED1.5 values >190 Gy. The reported incidence of late Grade 3 GU and GI toxicity was 3.1 and 0.4 %, respectively. In a scheme consisting of EBRT interdigitated with BRT, Prada et al. (2012) reported on 252 high-risk patients treated with 46 Gy EBRT and two fractions HDR BRT (on day 5 and 15 of EBRT) of 10.5–11.5 Gy yielding total combined BED1.5 doses ranging from 292 to 366 Gy. The accomplished 5- and 10-year BC rate was 84 and 78 %, respectively, with 2.7 % late Grade 3 GU and no late GI toxicity.
Even though a variety of clinically implemented dose schedules make uniform recommendations concerning the optimal combined dose regime difficult, the literature data on HDR BRT with supplemental EBRT are consistent and reproducible (Table 3). The high clinical efficacy of the so-called “combined treatment protocols” is explicable by the higher dose that can be prescribed when BRT is used for treatment and by virtue of a higher dose within the implant. Most authors use BRT fractions ranging from 6 to 10 Gy yielding total physical HDR doses of 12–20 Gy applied in two to four fractions. The supplemental EBRT doses range from 45 to 54 Gy, generating total BED1.5 and EQD2 doses in the range of 171–366 Gy and 74–137 Gy, respectively (Galalae et al. 2002, 2004, 2006; Pistis et al. 2010; Kotecha et al. 2013; Prada et al. 2012; Martinez et al. 2002, 2003, 2005, 2011; Demanes et al. 2005; Sathya et al. 2005; Vargas et al. 2006; Martin et al. 2004; Aström et al. 2005; Hiratsuka et al. 2004; Noda et al. 2011; Deger et al. 2005; Morton et al. 2010; Agoston et al. 2011; Izard et al. 2006; Pellizzon et al. 2008; Phan et al. 2007; Viani et al. 2009). The reported rates of late Grade 3 GU adverse events are far less than 5 % in the majority of HDR boost series comparing favorably with high-grade late GU toxicity rates in modern dose-escalating EBRT series (Peeters et al. 2006; de Meerleer et al. 2004; Hanks et al. 2000).
Overall, HDR BRT with supplemental EBRT enables the highly conformal administration of escalated biological doses to the prostate while ensuring favorable toxicity profiles. At this point, there is no consensus about the role of androgen deprivation therapy (ADT) in combination with HDR BRT for the treatment of locally-confined prostate cancer. Temporary hormonal ablative treatment may not be of additional advantage since it has not shown to be associated with improved oncological outcomes (Galalae et al. 2004; Demanes et al. 2009; Hoskin et al. 2007; Martinez et al. 2003, 2005).
4.2 HDR Monotherapy
Mature results from different institutions demonstrate HDR monotherapy to be an excellent option for the definitive treatment of organ-confined disease. Constant and reproducible 5-year BC rates have been reported for patients with low-risk (85–97 %), intermediate-risk (93–97 %), and high-risk (79–88 %) prostate cancer (Zamboglou et al. 2012; Demanes et al. 2011; Hoskin et al. 2012; Yoshioka et al. 2011, 2013; Rogers et al. 2012; ; Corner et al. 2008; Ghilezan et al. 2011; Martin et al. 2004; Mark et al. 2010; Barkati et al. 2012; Grills et al. 2004; Martinez et al. 2001, 2010; Komiya et al. 2013; Ghadjar et al. 2009; Prada et al. 2012) using a variety of dose-fractionation regimes. Table 4 lists dose-fractionation schemes and associated BEDs of monotherapy protocols from the literature.
Table 4
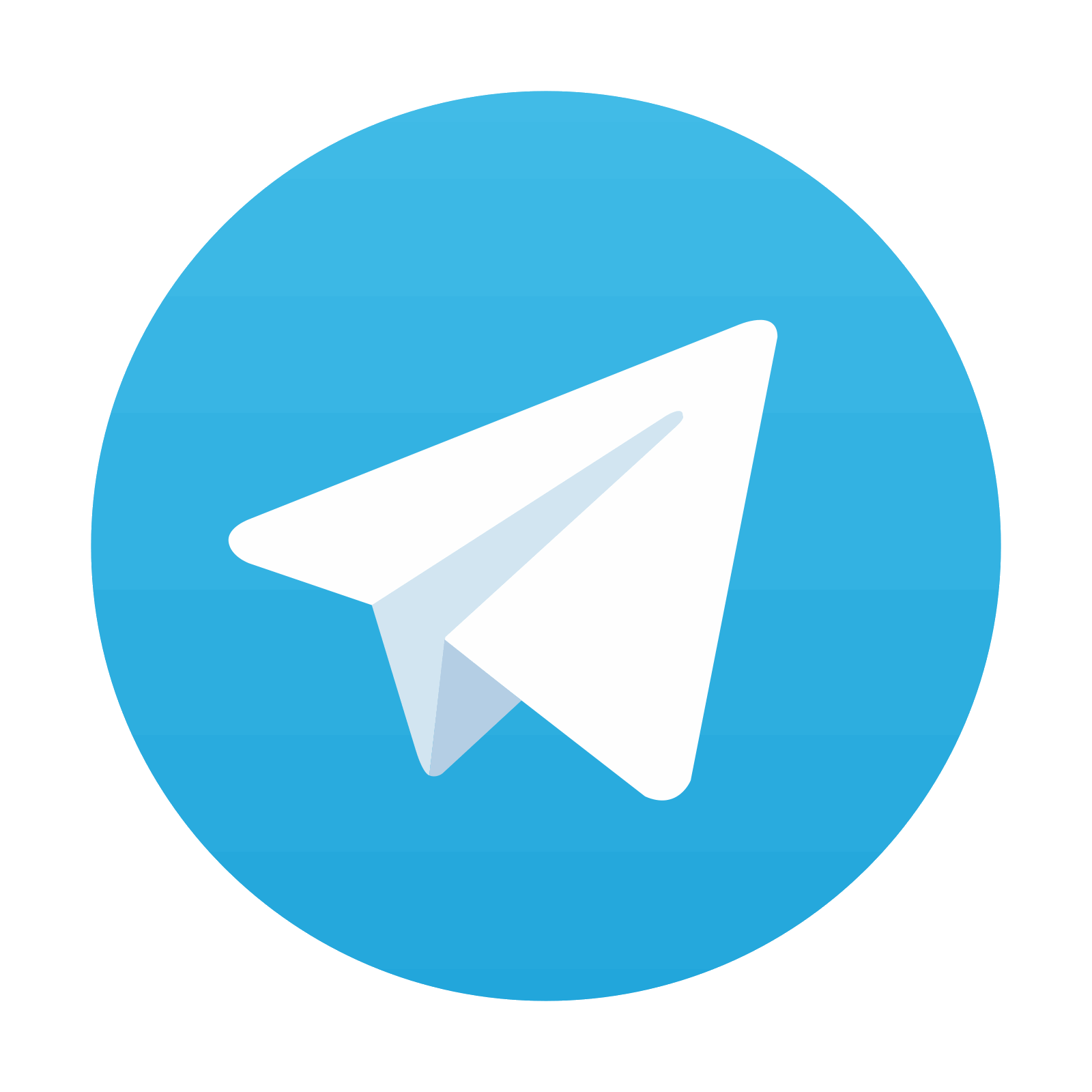
Literature results of high-dose-rate brachytherapy as monotherapy for localized prostate cancer
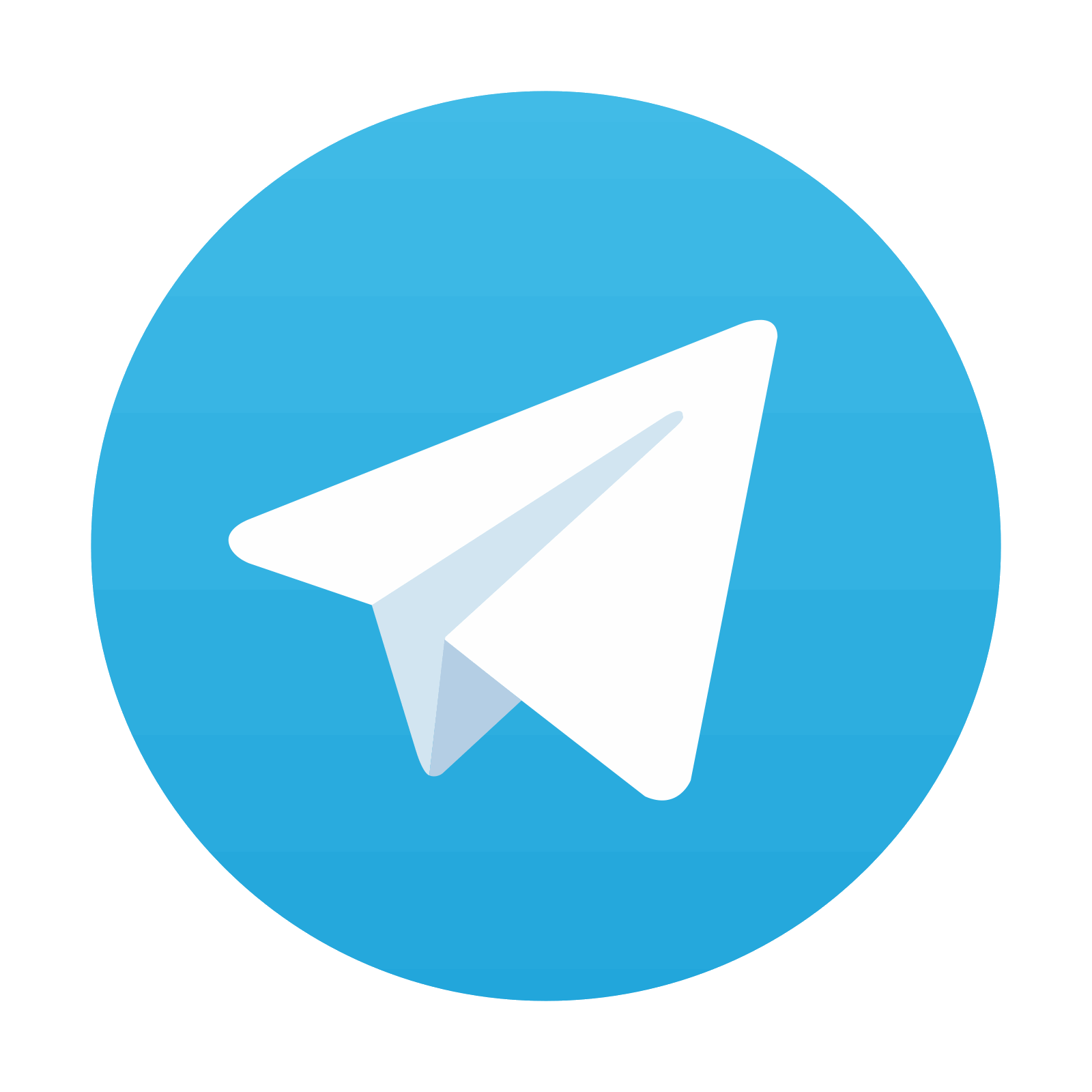
Stay updated, free articles. Join our Telegram channel
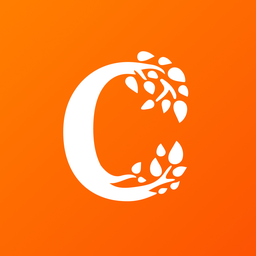
Full access? Get Clinical Tree
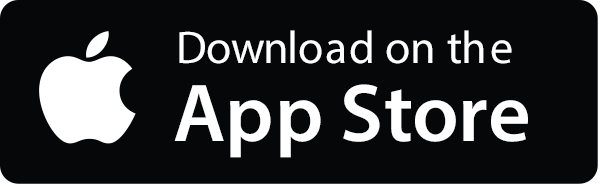
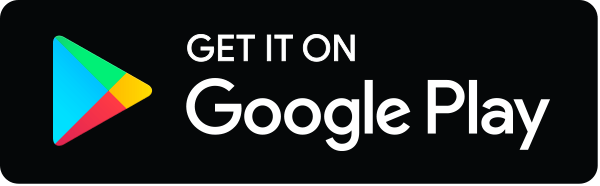
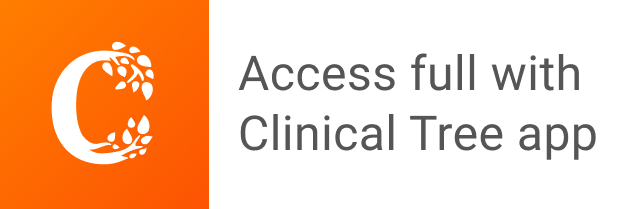