Fig. 9.1
Integrated PET/CTA study in a 66-year-old man with obesity, dyslipidemia, and diabetes mellitus. (a) Curved multiplanar reconstructions. The proximal LAD shows a long calcified plaque with 85 % stenosis. The LCx artery shows calcified plaques with 47 % stenosis proximally and 77 % stenosis distally. The RCA has a proximal, calcified plaque with 92 % stenosis. The summed stenosis score was 19 in the LAD, 6 in the LCx, and 16 in the RCA. The Duke CAD index was 74. (b) Stress and rest myocardial perfusion imaging shows extensive and severe ischemia in LAD territory with transient ischemic dilatation. (c) PET/CTA fusion imaging demonstrating that the mid- and basal inferior segments were supplied by a large-dominant LCx in contrast to the standard AHA model. (d) MFRregional were 0.57, 1.72, and 1.05 in the in LAD, LCx, and RCA territories, respectively. MFRglobal was 1.28 (Adapted with permission from Naya et al. [85])
Radionuclide perfusion imaging provides information about myocardial perfusion; however, it lacks information about coronary anatomy. Hence, nonobstructive stenoses below the threshold of causing an abnormal perfusion deficit cannot be detected. Simultaneous co-registration of perfusion deficit to a coronary stenosis and coronary artery distribution remain a challenge in the absence of information on individual coronary anatomy. Standardized allocation of myocardial segment to the individual coronary artery and mental co-registration during scan interpretation may be a simplistic and familiar approach; however, significant anatomic variation exists among standardized vascular “territories” and corresponding coronary segments resulting in disagreement in up to 72 % of the cases [26]. Also, microvascular dysfunction in the absence of epicardial stenosis cannot be ascertained. Thus, accurate spatial localization of myocardial perfusion deficit and designating the individualized coronary artery segment significantly improve the diagnostic value of hybrid imaging and help in devising preventive and therapeutic intervention [17, 27].
9.2.2 Myocardial CTP Imaging
Myocardial CT perfusion imaging was made feasible with the availability of 16-row detector scanner systems [28, 29]. Progression of CT technology to 64-slice scanners allowed the combination of reliable coronary angiogram with stress-induced myocardial CTP technically possible to perform. Previous studies have shown good results with 64-row single-source and dual-source CT scanners [30–33]. However, these systems provide limited coverage of the heart and require cardiac volume acquisitions over several heartbeats, resulting in the more cranial portions of the heart being scanned earlier in time than the more caudal portions causing temporal heterogeneity and making comparisons in signal intensities between the two areas more difficult due to time-related changes in the myocardial contrast concentration [34, 35]. Introduction of wide-coverage (256-row and 320-row) MDCT systems permitted coverage of the entire cardiac volume in a single heartbeat over one gantry rotation. Along with the wide-coverage scan acquisition, prospective cardiac-gated imaging triggered during a specific period of the cardiac cycle (static CTP) mitigated the problem of temporal heterogeneity caused by time attenuation of iodinated contrast concentration in the myocardium. This resulted in significant reduction in radiation so as to permit combined coronary CTA and stress myocardial perfusion assessment, providing comprehensive cardiac assessment with acceptable radiation dose. Additionally, faster acquisition has decreased scan times, reducing the amount of contrast material per scan [36].
Contrary to radionuclide myocardial perfusion imaging that uses tracers tagged with radioisotopes, myocardial CTP utilizes iodinated contrast as a perfusion agent. By measuring the concentration over time of the tracer in the myocardium, absolute blood flow in different regions of the myocardium can be estimated [34]. Iodinated contrast has comparable pharmacodynamics properties to gadolinium-based contrast agent used for magnetic resonance perfusion imaging. Iodinated contrast agent is extracted into the extracellular space in a linear relation to time and is excluded from the intracellular space bound by intact cellular membranes [37]. This concentration of iodinated contrast is directly proportional to the measured signal attenuation on a CT image (Hounsfield unit) (Fig. 9.2a, b). It represents a linear relationship between CT-derived metrics and myocardial blood flow and volume, which can be quantitatively analyzed [30, 34]. The extraction fraction of iodinated contrast from intravascular into the extracellular space is paradoxically higher at low blood flow and low at high flows [37]. This is important to consider when quantifying myocardial blood with CT, particularly in regard to correcting for the extraction of iodinated contrast at various flows.
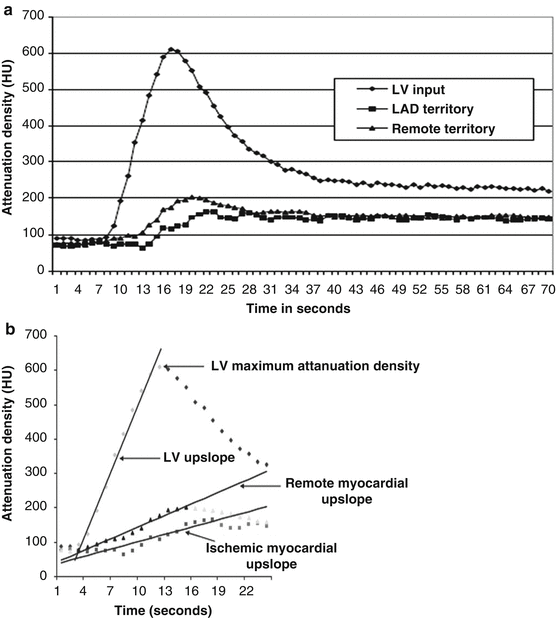
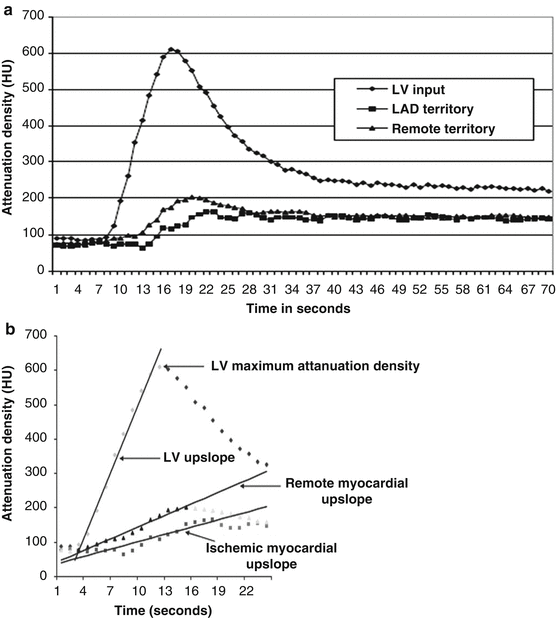
Fig. 9.2
(a) Time-attenuation curves for the left ventricle (LV), left-anterior descending artery (LAD), and remote territories. Attenuation densities over time duration are expressed in Hounsfield units (HU). Significant differences are noted in the time-attenuation curves between stenosed LAD territory and remote myocardium. Also note that the LAD territory curve has a delay in onset and time to peak contrast enhancement as well as a lower peak myocardial attenuation density. (b) Dynamic MDCT parameters used in the upslope analysis methods. Upslopes of the left ventricular (LV) blood pool, remote myocardium, and ischemic myocardium were calculated by applying a best-fit linear regression line from the data points when iodinated contrast first enters the LV cavity to the peak contrast enhancement of the region of interest. Myocardial upslopes were normalized to the LV maximum attenuation density (MUS/LVM ratio) and the LV upslope (MUS/LVUS ratio) (Adapted with permission from George et al. [34])
Myocardial rest CT perfusion and delayed enhanced CT are capable of defining myocardial tissue characteristics [38, 39] as well as detecting acute and chronic myocardial infarction and viability [40–42]. However, for the detection of myocardial ischemia, a provocative stress test is necessary to assess for inducible myocardial ischemia. This can be performed by using exercise or dobutamine, both resulting in an increase in heart rate, or using pharmacologic vasodilation with adenosine, dipyridamole, or regadenoson. Since increase in heart rate is not ideal for quality cardiac CT scan acquisition, a vasodilator drug is a preferred stress agent for myocardial CTP with stress images acquired at the time of maximum hyperemia [30, 31, 34]. Adenosine is usually employed as a vasodilator agent and has the advantage of a short half-life that precludes need of reversal agent. However, adenosine administration requires an infusion pump, and side effects include atrioventricular block and bronchospasm [30, 31, 43]. Dipyridamole shares similar pharmacologic properties and side effect with adenosine except having a longer half-life that often requires reversal with aminophylline. Regadenoson is a specific A2A agonist with lower incidence of side effects, primarily in regard to bronchospasm and heart block. Regadenoson’s greatest advantage is its ease of administration. It comes in a prefilled syringe with uniform dosing for all patients eliminating drawing up or mixing of drug, infusion pump, and tubing. Regadenoson does cause a slightly higher heart rate than adenosine and dipyridamole [44, 45].
Myocardial stress CTP images can be acquired during a specified time period around the peak of the contrast bolus (static imaging) or serially as the contrast bolus traverses the coronary arteries and myocardial vasculature (dynamic perfusion). For static CTP, images are acquired during the late upslope, peak, and early downslope (Fig. 9.2a) of the contrast bolus and provide data that are qualitative or semiquantitative [34]. Dynamic CTP involves construction of time-attenuation curves of the myocardium and the reference artery allowing calculation of myocardial blood flow and flow reserve [34, 46].
9.2.3 Computed Tomography-Fractional Flow Reserve (CT-FFR)
Recent developments in image-based modeling techniques have allowed accurate segmentation of coronary artery tree and application of computational fluid dynamics within the confines of coronary artery system. Mediated by complex algorithms and calculations applied to the simulated boundary conditions, coronary flow and pressure information can be obtained from rest CTA images. These models can also be used to quantify luminal stenosis and perform flow-pressure simulation models to determine the hemodynamic significance of a lesion, without a necessity of additional scan acquisition or administration of a pharmacologic stress agent.
The CT-FFR method involves derivation of fractional flow reserve (FFR) from anatomic CT data. FFR is defined as a ratio of intracoronary pressure distal to a stenosis and aortic pressure. Principally, segmentation algorithms are applied to create an anatomic model of the coronary artery anatomy followed by mathematical representation of coronary physiology derived using cardiac output, aortic pressure, and microcirculatory resistance. Allometric scaling laws, which analyze the relationship of mass of an object to shape, anatomy, and physiology, are used to define the relation of organ size to flow rate [47]. This enables the computation of coronary flow and pressure. Exploiting these principles, myocardial mass and volume extracted from the coronary CTA data are applied to estimate overall coronary flow under resting conditions. Based on consistent relationship between coronary flow and resistance, total coronary resistance can then be calculated using the coronary flow rate. Extending the mathematical relationship between vessel size and flow rate based on Poiseuille’s equation (Q f(dk), where Q is the flow rate through a blood vessel, d is its diameter, and k is a constant), information on the flow rate and relative resistance of the branches arising from the coronary arteries are obtained (Fig. 9.3).
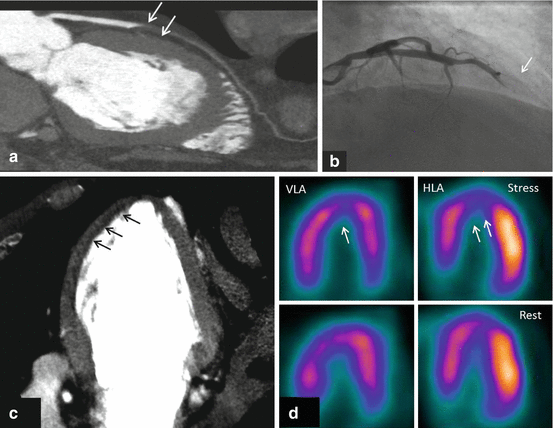
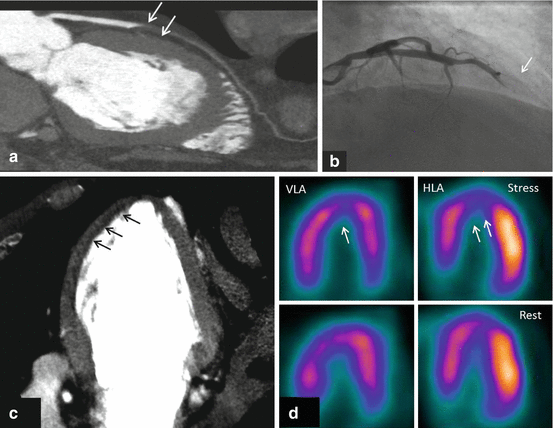
Fig. 9.3
Figures represent combined coronary CTA and myocardial CTP imaging showing flow-limiting stenosis confirmed by invasive coronary angiography and SPECT. CTA (panel-a) shows total occlusion of mid-LAD with corresponding CT hypoattenuation (panel-c, black arrows) involving anterior, anteroseptal, and apical myocardial segments consistent with myocardial CTP deficit. Panel b shows complete occlusion (100 % lesion) of mid-LAD on invasive coronary angiography with concurrent SPECT perfusion (panel d) defects read in similar myocardial segments as CTP
Image segmentation algorithms define the luminal surface of the major vessels and branches, leading up to the vessel diameter limited by the spatial resolution of CTA. Based on the morphometric law, a unique resistance value is then assigned for each coronary artery and its branch outlets. Finally, maximum hyperemic conditions are simulated to model in the effect of adenosine administration (76 % drop from the resting values with intravenous administration of 140 mg/kg/min adenosine) [48] in decreasing the coronary resistance (Fig. 9.4). CT-FFR can then be determined by solving the equations of blood flow for the velocity and pressure fields and normalizing the mean hyperemic pressure field by the average mean hyperemic pressure in the aorta.
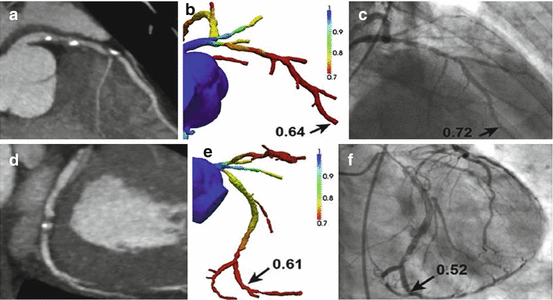
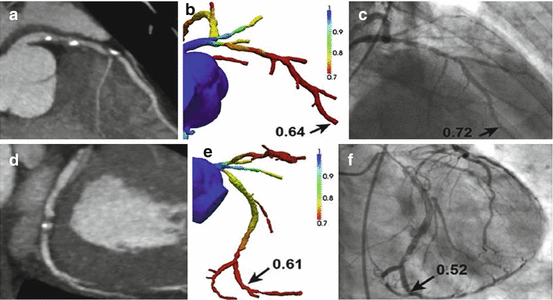
Fig. 9.4
(a) CTA demonstrating stenosis in the LAD. (b) FFRCTA demonstrates ischemia in the LAD, with a computed value of 0.64. (c) ICA with FFR also demonstrates ischemia in the LAD, with a measured value of 0.72. (d) CTA demonstrating stenosis in the LCx. (e) FFRCTA demonstrates ischemia in the LCx, with a computed value of 0.61. (f) ICA with FFR also demonstrates ischemia in the LCx, with a measured value of 0.52 (Adapted with permission from Taylor et al. [13])
9.3 Clinical Evidence
9.3.1 Hybrid Imaging
With technical enhancements in image processing software and advent of hybrid scanners, it is possible to perform fusion of the two imaging modalities with simultaneous co-registration of anatomic-physiologic information that enables assigning perfusion territories to a specific stenosis. Clinical data on the diagnostic utility of hybrid imaging is largely restricted to single-center studies. Among intermediate- to high-pretest-likelihood patients, Schaap et al. reported that hybrid SPECT/CTA had a sensitivity of 96 %, specificity 95 %, PPV 96 %, and NPV 95 % [49]. The same group, in a high-risk and known CAD population, also reported that hybrid SPECT/CTA imaging had better diagnostic performance (c-index, 0.96) compared to SPECT (0.85) and CCTA (0.90) alone [50]. Another study integrating SPECT and CTA stated PPV of 77 % compared to 31 % for CTA alone in detecting myocardial ischemia [51]. Sato and colleagues [52] reported augmentation of PPV from 69 to 85 % by combining SPECT information to non-evaluable coronary arteries on CCTA.
In a study of 107 patients, H2 15O PET/64-slice CTA had a diagnostic accuracy of 98 % with significant improvement in PPV of 96 % for hybrid imaging compared to 76 % for CTA alone [53]. The reference standard was ICA with FFR ≤0.80 when feasible. Another study confirmed these findings with reported sensitivity and specificity of 98 % and 91 %, respectively [54]. Danad et al. [55] attested the “gatekeeper” role of hybrid imaging for downstream revascularization and referral for ICA. Of 5 % of the patients with “normal” CTA that were referred for invasive angiography, none had significant CAD. Among the patients that had an equivocal CTA result, 18 % with normal PET-MPI were referred for ICA compared to 71 % in abnormal MPI group, though no coronary revascularization was done in the normal PET-MPI group. Similarly, 72 % patients underwent revascularization for concurrent CTA stenosis and abnormal perfusion versus 26 % with obstructive stenosis (≥50 %) on CTA but normal PET imaging. Hybrid imaging had incremental diagnostic utility in 29 % of the patients, particularly influencing individuals with multivessel disease and/or intermediate-grade stenosis. Thus, combined anatomic-physiologic information derived from hybrid imaging, particularly in the setting of equivocal functional imaging, has been of clinical significance leading to the proportionate decline in false-positive testing. This improved accuracy was most relevant in the segments perfused by the left circumflex and right coronary artery, affirming the inconsistencies that exist among the myocardial segments perfused by respective coronary vessel [56].
9.3.2 Myocardial CT Perfusion
Numerous single-center studies initially established the diagnostic efficacy of CTP in assessing the flow-limiting stenosis. Using 64-row and 256-row MDCT scanner systems and defining the gold standard as a combination of quantitative coronary angiography and concurrent perfusion abnormalities by SPECT, George et al. on per-patient basis reported the sensitivity, specificity, PPV, and NPV of 86 %, 92 %, 92 %, and 85 % for combined CTA and CTP, respectively. For per-vessel/territory analysis, these corresponding values to detect obstructive stenosis were 79 %, 91 %, 75 %, and 92 %, respectively [30]. Blankstein et al. showed that CTP alone had a sensitivity of 93 % and a specificity of 74 % for the detection of vessels with at least 50 % stenosis and a corresponding SPECT perfusion abnormality [31]. Myocardial CTP has also been compared to invasive FFR in the single-center study by Bettencourt et al. [57] This study enrolled 101 symptomatic patients at intermediate to high risk for CAD. When compared to invasive FFR, myocardial CTP has a diagnostic accuracy of 85 % and a sensitivity, specificity, PPV, and NPV of 89, 83, 80, and 90 %, respectively. Radiation dose was quite low at 5.0 ± 0.96 mSv for the CTA and CTP study combined. Another single-center study examined the added value of CTP over CTA in the diagnosis of in-stent restenosis. Studying 91 patients with suspected in-stent restenosis, Rief et al. [58] tested the diagnostic performance of CTA alone and in combination with CTP. Compared to CTA alone, nondiagnostic rates dropped from 20 to 0 %. There were significant improvements in accuracy, sensitivity, and specificity. Overall diagnostic performance measured by the AUC of the receiver-operator characteristic curve was 0.76 for CTA alone and 0.90 for CTA + CTP (p < 0.001).
Following the encouraging results from the single-center experiences, the coronary artery evaluation using 320-row multidetector computed tomography angiography and myocardial perfusion study (CORE320) was an international, multicenter study aimed to establish the incremental diagnostic utility of CTP over CTA against the reference standard of invasive coronary angiography and a corresponding perfusion deficit on SPECT-MPI. The study included patients with prior intracoronary stents and prior myocardial infarction. The study enrolled 381 patients and demonstrated a sensitivity of 94 % and specificity of 64 % for the presence of ≥50 % stenosis by CTA alone to predict the reference standard of combined ICA-SPECT with an AUC (0.84, 95 % CI: 0.79–0.88). The study confirmed significant increase in the patient-based diagnostic accuracy when CTP was added to the CTA (AUC 0.87, 95 % CI: 0.84–0.91). The AUCs for combined CTA-CTP imaging continued to rise to 0.90 for patients without prior myocardial infarction and 0.93 for patients without prior CAD. For the combination of a CTA stenosis ≥50 % stenosis and a CTP perfusion deficit, the sensitivity, specificity, PPV, and NPV values were 80 %, 74 %, 65 %, and 86 %, respectively [12]. A subsequent multicenter study verified that CTP had a good agreement with SPECT (87 %) imaging and the diagnostic accuracy of CTA (0.69) for detecting a reversible perfusion defect on SPECT improved to 0.85 with the combination of CTP [59]. This study demonstrated that myocardial CTP is non-inferior to SPECT myocardial perfusion imaging for the detection of ≥2 segmental reversible perfusion deficits. Interestingly, CTP has been noted to have a higher sensitivity than SPECT-MPI for detecting of multivessel disease [60].
9.3.3 CT-FFR
CT-FFR derived using rest CTA images has been shown to have improved diagnostic accuracy in predicting lesion-specific ischemia, as determined by invasive FFR. In the Discover-Flow study, Koo et al. [61] in a per-vessel analyses reported better diagnostic accuracy of CT-FFR (AUC, 0.84) compared to CTA stenosis of ≥50 % (AUC, 0.59). On a per-patient basis, CT-FFR had an AUC of 0.87 with stated sensitivity of 94 %, specificity of 82 %, NPV of 0.91, and PPV of 0.85, respectively. In follow-up to the Discover-Flow trial, DEFACTO studied 252 patients with CT-FFR compared to the reference standard invasive FFR. CT-FFR did improve diagnostic accuracy from 64 to 73 %, but overall the trial did not meet its non-inferiority margin. More recently, the Analysis of Coronary Blood Flow Using CT Angiography: Next Steps (NXT) trial evaluated the diagnostic performance of updated iteration of CT-FFR utilizing invasive FFR as a reference standard [14]. In this study, a rigorous quality control mechanism was put in place for CTA and invasive FFR. Of the 365 subjects enrolled with a clinical indication for ICA, 251 subjects made it into the final analysis with 103 studies rejected due to CTA or FFR quality issues. In this study, CT-FFR – on per-patient basis – had an improved diagnostic accuracy with AUC of 0.81 versus 0.53 of CTA alone for detecting flow-limiting stenosis by invasive FFR. CT-FFR had better per-patient sensitivity of 86 %, specificity of 79 %, PPV of 65 %, and NPV of 93 % compared to CTA with corresponding values of 94 %, 34 %, 40 %, and 92 %, respectively. In a meta-analyses to assess the diagnostic performance of CT-FFR, Li and coauthors [62], in a per-patient analysis, reported pooled sensitivity of 89 %, specificity of 71 %, PPV of 70 %, and NPV of 90 % to predict the gold standard of invasive FFR ≤ 0.8. At the vessel level, there was improvement in diagnostic performance of CT-FFR with pooled specificity of 78 % and a comparable sensitivity of 83 %, respectively [62]. Overall, CT-FFR appears to be a robust method to improve the accuracy of CTA to predict the hemodynamic significance. However, CT-FFR has not been extensively tested in patients with prior revascularization or prior infarcts.
9.4 Limitations
Hybrid imaging has significant implications in terms of additional cost, contrast, and radiation exposure. An additional scan may also confer diagnostic delays and logistic challenges that significantly add to patient anxiety and inconvenience. Therefore, concerted efforts are being made to minimize these problems. Recent years have witnessed significant advances in imaging technology to decrease radiation dose. For SPECT-MPI, introduction of novel small-footprint cardiac scanners armed with solid-state detectors [63] and performance of half-dose perfusion imaging [64] have led to significant reduction in radiation exposure. Optimizing diagnostic yield by using high-efficiency cameras and employing stress-first protocols in individuals with predefined low-intermediate CAD risk have pushed the radiation doses for SPECT imaging to as low as 2.2 mSV [65]. The PET perfusion tracers (i.e., H2 15O, 13NH3, and 82 Rb) construe low radiation doses (1–2 mSv) and may be advantageous for hybrid imaging [66]. Recent advances in CT technology with emphasis on dose reduction techniques like limiting scan length, ECG-gated dose modulation, prospective ECG-triggering, and high-pitch scanning protocols have resulted in significant reductions in effective radiation dose [67]. Additionally, post-processing methods such as iterative reconstruction [68] and availability of next-generation scanners with faster gantry rotation time and wide volume coverage have implied substantial reduction in the amount of radiation during diagnostic testing. With current strategies, a hybrid stress-only SPECT/CTA or PET/CTA studies could be performed with radiation exposure less than or equivalent to invasive diagnostic angiography [69]. Availability of PET perfusion tracers limited by on-site cyclotron is a significant hindrance for performance of PET/CTA hybrid imaging. Although [81] Rb is a generator-based tracer, it requires large patient flow for its commercial viability. Industrial delivery of perfusion tracers with longer half-lives similar to fluorodeoxyglucose (18F) may help alleviate this problem.
MDCT imaging may be influenced by problems arising from image artifacts, lower contrast resolution, contrast dose, and optimal contrast timing. Imaging artifacts are an inherent limitation to CT technology with particular references to artifacts arising out of cardiac motion, beam-hardening, and reconstruction artifacts [70, 71]. Motion artifacts can be further incited by vasodilator stress agents that increase the average heart rate by 20–25 beats/min. These motion artifacts could be avoided by using β-blocker medications and improving the temporal resolution of the scan by using multicycle reconstruction or dual-source CT. Availability of wide-area multidetector scanner that allows coverage of entire cardiac volume in a single gantry rotation has considerably mitigated this problem [30, 32]. Beam-hardening artifacts are similar to attenuation artifacts in SPECT and arise from the high-attenuation structures (bones and contrast filled ventricular cavity and vessels) that absorb low-energy photons in proximity with the myocardium resulting in low-attenuation streaks. This can be negated by using corrections built into the reconstruction algorithms and by accounting for these artifacts during interpretation of the scan [71, 72].
Strict adherence to imaging protocols to ensure optimum image quality is critical for CT-FFR assessment. CT-FFR requires accurate anatomic framework derived using the image segmentation algorithms that can be influenced by image quality. Hence, the artifacts may have an amplified effect on the calculation of CT-FFR. The presence of coronary calcification and/or stents may result in misrepresentation of CT-FFR. Additional limitations of CT-FFR relate to physiological assumption models that include population as well as patient-specific data. Deduced relationships relating myocardial mass to total coronary flow, the relative coronary microvascular resistance calculated based on vessel size, or reduction in vascular resistance in response to adenosine-mediated hyperemia will not be consistent among all individuals. Extrapolation of these assumptions to calculate lesion-specific ischemia involves certain degree of variation.
While the results of integrated imaging modalities in assessing lesion-specific or patient-specific ischemia are promising, the data from the published studies are limited and lack uniform reference standard. The applicability and usefulness of combined imaging modalities have not yet been tested beyond clinical trials in the real-world clinical scenario. Currently, there are no data on the cost-effectiveness and improvements in patient outcomes as well as which patient subset will benefit from integrated imaging accounting for cost and radiation dose.
9.5 Prognostic Value
The presence of obstructive CAD and corresponding perfusion defect has a synergistic effect on incidence of adverse cardiovascular events; however, the presence of either conveys an independent prognostic risk. Consistent with this principle, a number of studies have documented improved efficiency of hybrid imaging in predicting adverse cardiovascular risk [11]. In a single-center study of 335 consecutively enrolled patients, Pazhenkottil and coauthors [11] stratified the patients based on CTA stenosis and the presence or absence of matching SPECT perfusion deficit. Combination of abnormal CTA-SPECT translated into worst prognosis with annual major adverse cardiovascular event rate of 21.0 and 6.0 % incidence of death/myocardial infarction. Normal dual-modality scan had lowest major event rate of 2.2 % with 1.3 % risk of death or coronary events. Patient with CTA stenosis and discordant result on MPI had intermediate major adverse event rate of 7.8 % and hard event rate of 2.8 %, respectively. Van Werkhoven et al. [73] had similar findings with annual event rate of 1.0 % (death/MI 0.6 %) in patients with normal CTA (<50 % stenosis) and a normal SPECT-MPI, whereas adverse event rate in patients with obstructive CAD (≥50 %) and corresponding SPECT perfusion abnormality was 9.0 % (death/infarction = 6.0 %). These findings are currently limited to single-center data with relatively smaller cohort size.
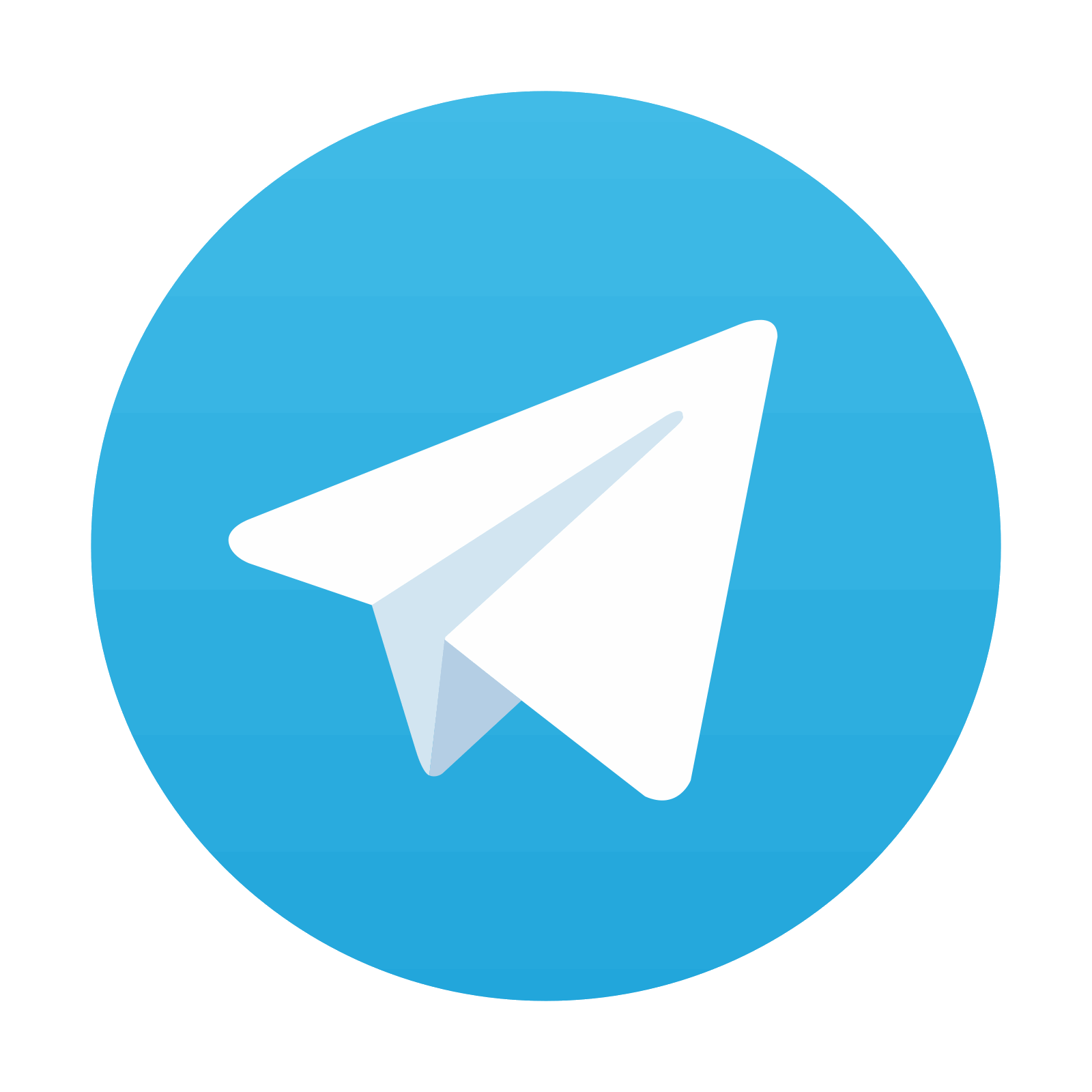
Stay updated, free articles. Join our Telegram channel
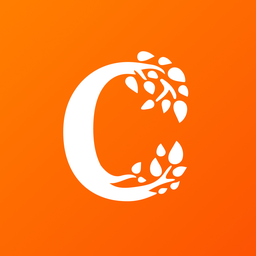
Full access? Get Clinical Tree
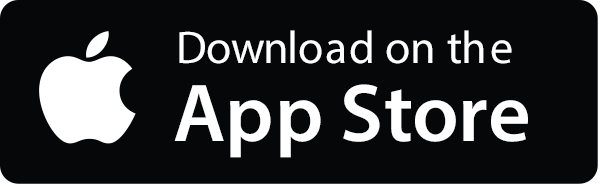
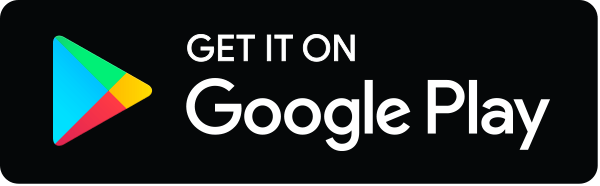