Fig. 21.1
Schematic diagram of the scattering and/or stopping of muons and electrons in different materials. Particle scattering and stopping increases as the density and the atomic number of the material increases
21.3 MMPDS Hardware
DSIC has developed and manufactured several scanners of different configurations to demonstrate the capabilities of muon tomography for the detection of concealed SNM. In addition to muon tomography, the scanner uses passive gamma detection to detect concealed radioactive objects. Two large scale systems have been built, as well as two smaller systems intended for research applications. The system in Freeport (referenced earlier), is capable of scanning full-size trucks and shipping containers (40 ft and larger) for concealed SNM materials. The second system, based at the DSIC facility in Poway, CA, is used for testing and optimization of the detector. The two research systems were delivered to commercial customers.
The sealed drift tube is used as the primary sensor element in the scanner (Fig. 21.2).5 A drift tube is an ionization detector that produces electrical signals in response to ionizing particles or radiation that pass into or through its volume. Sealed drift tubes are very robust, durable sensors requiring no maintenance over many years. Each tube can provide high-precision position measurement of a traversing charged particle over a large area while requiring only one signal processing channel. It is a sealed, gas-filled cylinder that has conducting walls (cathode) and a fine wire element strung longitudinally down the tube (anode). A high voltage is applied between the anode and the cathode. The gas in the drift tube mixture is ionized by the passage of muons or electrons which results in a number of electron-ion pairs (on the order of 25 pairs per 1 cm path of a muon). The drift-tubes comprising the scanner are also capable of detecting gamma rays. The incidence of gamma rays on the aluminum walls causes Compton electrons to be emitted from the conductive wall of the tube, causing ionization of the drift tube gas.
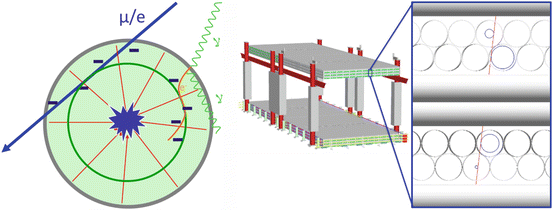
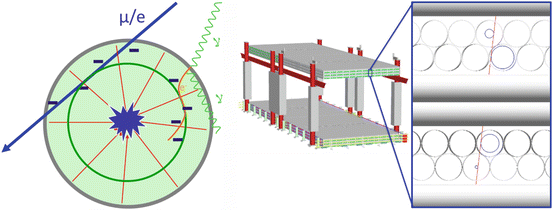
Fig. 21.2
Sealed drift tubes function by detecting ionization caused by the passage of charged particles through the gas volume. Using timing information of the arrival time of the ionized electrons, a radius can be calculated. This provides a sub-millimeter position resolution measurement of the path of the charged particle. The 3-D trajectory of the particle is reconstructed from measurements in several layers of tubes
The drift tube electrical signals are detected, amplified and identified by first stage electronics. The temporal information of the electrical signal can be used to determine the closest approach radius between the charged particle path and the wire. Custom electronics, installed at one end of the tubes, acquire, time stamp and filter tube signals. Data is delivered to an analysis cluster running software to identify muon and gamma events, calculate tracks and perform tomographic reconstruction that produces a 3-D map of the materials of the volume under inspection. Count rates on each tube are individually analyzed to produce a map of the position and distribution of radioactive sources within the volume.
The scanner is constructed using large arrays of sealed drift tubes, arranged in orthogonal layers, to enable 3-D tracking of muons and electrons. These arrays are arranged above and below the volume of interest and used to track each charged particle as it enters and leaves the detector (Fig. 21.3). Changes in the trajectory of the particle are analyzed to produce a 3-D representation of the materials in the volume. If the particle is attenuated in the volume, the absence of an outgoing trajectory is recognized and analyzed for the attenuation image.
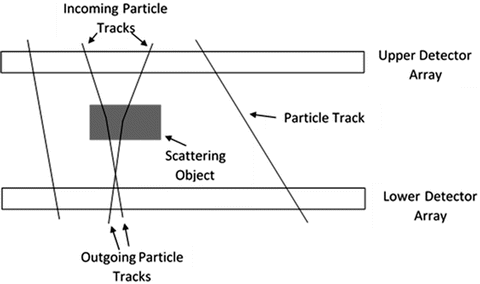
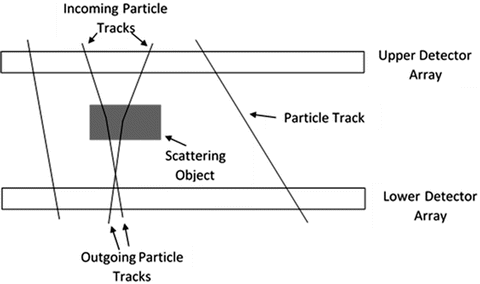
Fig. 21.3
Schematic diagram showing muons traversing the detector array and scattering in an object in the scan volume between the detector arrays. The scattering angle is greatly exaggerated in this figure; the actual angle is a few milliradians
21.4 Imaging and Detection Software and Algorithms
In the DSIC scanner, cosmic ray muons and electrons are tracked into and out of a volume of interest. Their collective scattering and attenuation information is then used to reconstruct the materials through which the muon and electrons have passed. Each scattered particle provides a measurement of the scattering angle and an approximate location of the scattering. These data are used to reconstruct a 3-D map of signatures related to the proton and electron densities of the interrogated materials. After tomographic reconstruction from particle scattering and momentum data, threat objects may be identified and distinguished from benign cargo on the basis of their size, shape, atomic number and density. For SNM and other high-Z, high density nuclear threats, muons are the primary probe, whereas for lower density contraband, including explosives and narcotics, a combination of muon and electron dynamics is most effective in detection and discrimination of the materials.
21.4.1 Charged Particle Imaging
The image reconstruction starts with determining the incoming and outgoing tracks of the muons and electrons as they pass through the top tracker, the scanned volume and the bottom tracker. The tracks are determined using the locations on the drift tubes at which the muons and electrons were incident. As stated above, this positional information can be derived from the temporal information of the electrical signal in the output of the drift tube (Figs. 21.4 and 21.5).
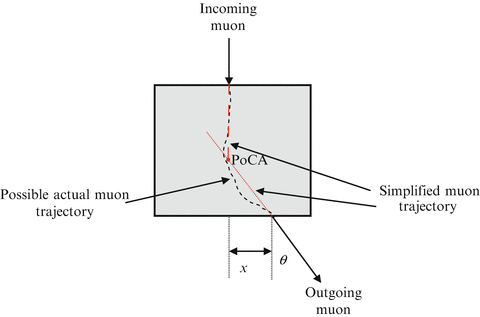
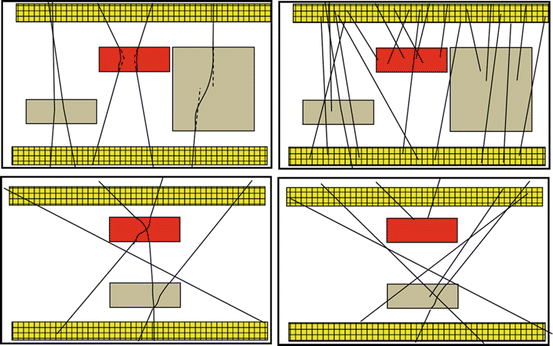
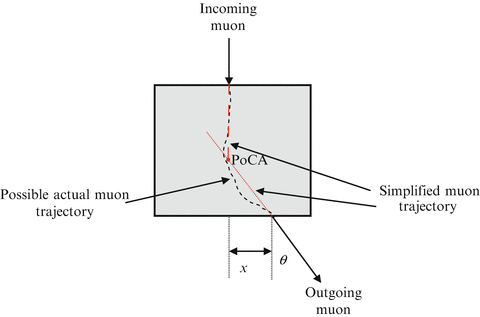
Fig. 21.4
Schematic diagram of the scattering of a cosmic ray particle in an object. The actual particle trajectory inside the object is not known, but the incoming and outgoing trajectory of the particle can be measured. The scattering angle is the angle θ between the incoming and outgoing particle trajectories. In a simplified model, the scattering location is considered to be in the region where the extrapolated incoming and outgoing trajectories are closest to each other (they will not necessarily intersect as shown in the figure). This location of closest approach is called the ‘point of closest approach’ or ‘PoCA’
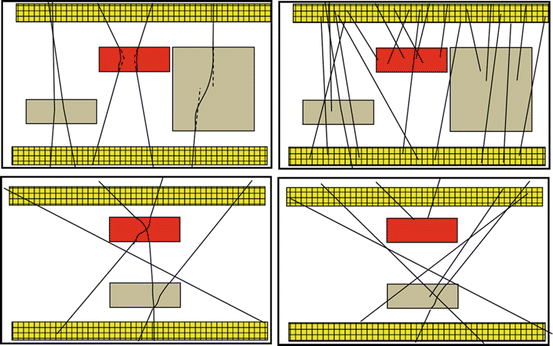
Fig. 21.5
Schematic diagram of scattering and stopping of tracks passing through objects in the scan volume between the upper and lower detector arrays (colored yellow). The scattering angle reflects the integrated proton density through which the particle passes. The scattering point provides information as to the vertical location of the scattering source. The distance of closest approach between the reconstructed trajectories provides information related to the physical thickness of the material traversed. Attenuated particles provide information related to the integrated stopping power of the material through which they pass. For both techniques, multiple angle exploration of the volume by charged particles helps to resolve remaining ambiguity as to the position of materials in the volume
21.4.2 Multiple Coulomb Scattering Imaging
The scan volume between the detector arrays is divided into voxels.6 Once the tracks are determined, the scattering angle θ and the scattering location and its corresponding voxel are estimated. This estimation varies based on the reconstruction algorithm used. A distribution is accumulated for each voxel. As more muons and electrons enter and scatter in the scan volume, the voxel scattering strength distribution is updated. The scattering density map is calculated at the end of a scan period using a statistic characterizing the mean square scattering of the distribution of scattering per unit depth within each voxel. Iterations of this process produce better estimates of the portion of scattering caused by each voxel and therefore higher fidelity estimation of the scattering density along the path of the charged particle.
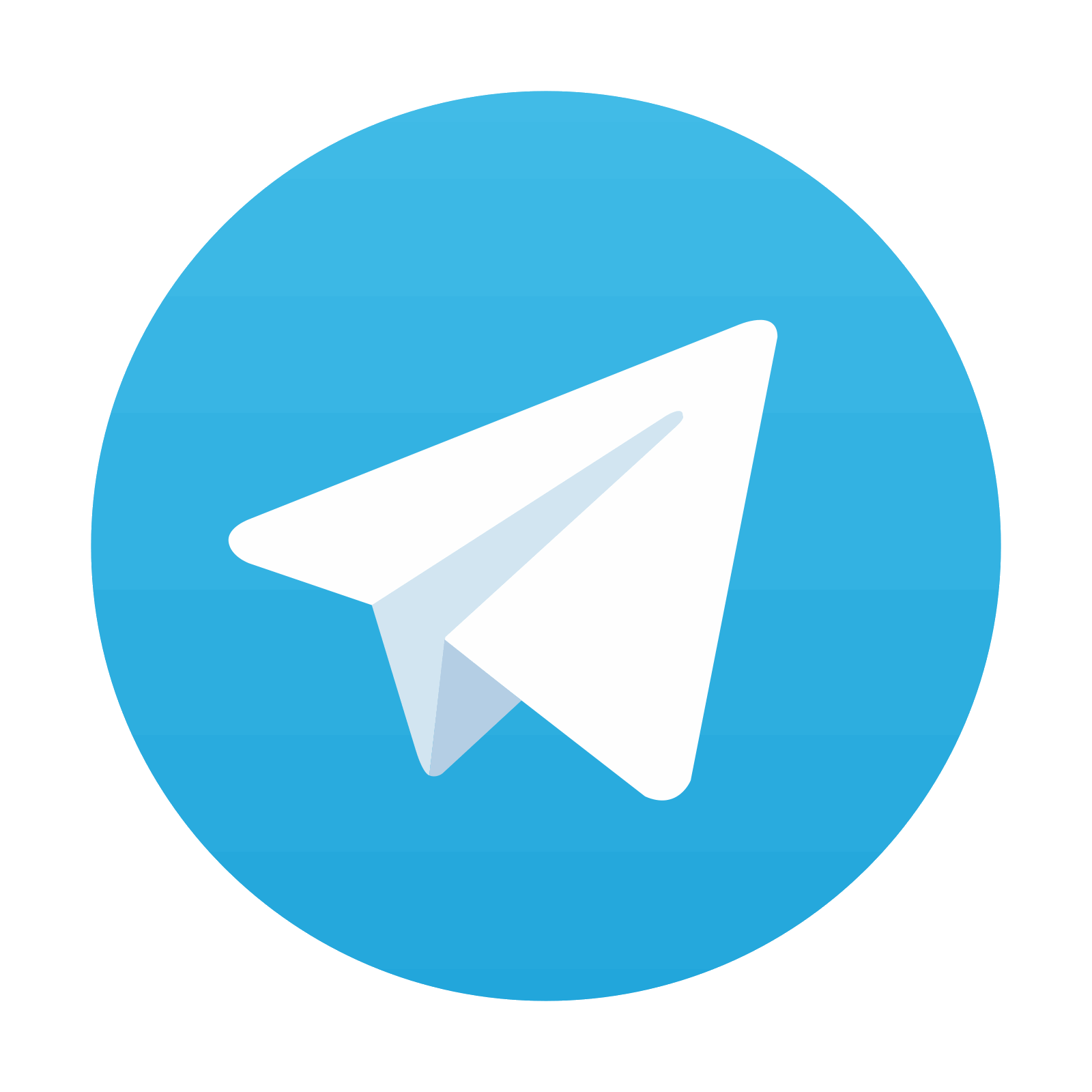
Stay updated, free articles. Join our Telegram channel
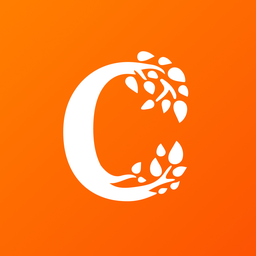
Full access? Get Clinical Tree
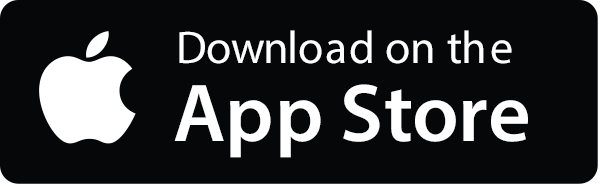
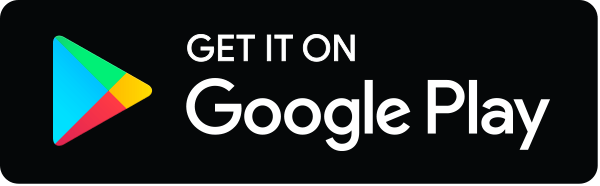