Fig. 1
In (a, b), the extravascular BOLD effect is associated with the magnetic field gradient generated outside the boundaries of the blood vessels, which is due to the difference in magnetic susceptibility induced by deoxyhemoglobin between the vessel and the surrounding diamagnetic tissue (after Hoppel et al. 93). The black curves outside the vessels represent the field gradient produced by the blood, which is inside the vessels. (a) For small vessels such as capillaries, the field gradients around the vessels are large compared to diffusion distances; therefore, the extravascular BOLD is a T2 effect as water diffusion dynamically averages in the magnetic field gradient. (b) For large vessels, the field gradients around the vessels are small compared to diffusion distances. The signal loss in the voxel is the result of a static averaging; therefore, the extravascular BOLD is a T2* effect. In (a, b), the intravascular BOLD effect is associated with the magnetic field gradient generated outside the red blood cells inside the vessels. Similarly to the extravascular BOLD effect around capillaries, the intravascular BOLD is detectable as a T2 effect
The intravascular T2-BOLD effect is associated with the magnetic field gradient generated outside the red blood cells and inside the vessels. The field gradients around the red blood cells are very small compared to diffusion distances around and across the membranes of these cells (Fig. 1). Therefore, the effect is dynamically averaged and is detectable as a T2 effect. When regional deoxyhemoglobin content increases, the T2 of blood decreases (Thulborn et al. 1982). This intravascular effect is present in large as well as in small vessels.
The extravascular BOLD effect is associated with the magnetic field gradient generated outside the boundaries of the blood vessels. This gradient is due to the difference in magnetic susceptibility induced by deoxyhemoglobin between the vessels and the surrounding diamagnetic tissue. For small vessels such as capillaries, water diffusion during image acquisition (typically 50–100 ms for echo planar images (EPI)) dynamically averages the magnetic field gradient and results in a T2 effect, similarly to the intravascular BOLD effect. For large vessels, dynamic averaging is not possible anymore. In contrast, static averaging will result in a signal loss within the voxel. A water molecule located in the vicinity of the blood vessel will see a static magnetic field, which will vary with the proximity to the vessel (Fig. 1). As this magnetic field varies across the voxel, the signal of the entire voxel will be dephased, resulting in a T2* effect.
1.1.2.2 Specificity of Spin Echo (T2) Versus Gradient Echo (T2*) BOLD
As detailed above, the T2-BOLD response arises from intra- and extravascular effects originating from small and large vessels. The intravascular contribution originates from both large and small vessels, whereas the extravascular part is dominated by small vessel contributions (Boxerman et al. 1995). Thus, the spin echo (SE) BOLD fMRI response is due to apparent changes in T2 originating from the diffusion of water in the presence of magnetic field gradients generated in the extravascular space around the microvasculature (Ogawa et al. 1993; Boxerman et al. 1995), as well as from the exchange of water into and out of red blood cells within the blood itself (Pawlik et al. 1981; van Zijl et al. 1998; Ugurbil et al. 1999). At high magnetic fields, blood has a short T2, shorter than the echo times that are used in fMRI experiments. Therefore, the blood is not expected to contribute significantly to the measured BOLD signal changes. Consequently, at high field strength, the SE BOLD mainly originates from the extravascular space around the microvasculature and hence provides greater specificity to changes in neuronal activity (Lee et al. 1999; Duong et al. 2003; Ugurbil et al. 2003; Yacoub et al. 2003).
1.1.2.3 Field Dependence of BOLD Signal
Both the signal-to-noise ratio (SNR) and spatial resolution of BOLD fMRI increase with the main magnetic field strength. Higher magnetic fields will also preferentially attenuate the macrovascular contribution (Ugurbil et al. 2003).
The BOLD response is expected to behave differently with increasing magnetic fields in small versus large blood vessels (Ogawa et al. 1993). The dependence on the external magnetic field strength varies quadratically for capillaries. In contrast, the dependence on the static magnetic field (B0) is linear for the contribution of large vessels (Ogawa et al. 1993). Therefore, in addition to increasing the fMRI signal, higher magnetic fields will specifically enhance the signal components originating from parenchymal capillary tissue. In contrast, BOLD signal originating from large vessels will be overrepresented at lower magnetic field strengths.
1.2 fMRI Artifacts and Limitations
Different types of artifacts and methodological limitations need to be considered in clinical fMRI. They may also interfere with the results of validation studies using established reference procedures – the most important are discussed below.
1.2.1 Movement Artifacts
Head motion is a critical issue in BOLD fMRI. Head motion is mostly reflected in rigid body global deformations and translations. When voxels have side lengths smaller than a millimeter, motion control becomes a crucial issue. Head motion does not depend on which part of the brain is imaged. Using single-shot EPI, each image is virtually devoid of motion artifacts, given the very short acquisition time (<100 ms). However, head motion may occur between successive images of one fMRI series. Most fMRI studies are currently performed with a spatial resolution of 3–4 mm (in-plane voxel size) and a slice thickness of 4–5 mm (e.g., matrix: 64 × 64, FOV = 24 cm). Head motion artifacts predominate in regions of tissue interface where changes in signal intensity are high (Fig. 2). When temporally correlated with task performance, a movement of one tenth of a voxel (300–400 μm) may result in signal changes similar or even larger than the actual signal increase due to the task performance and which are virtually impossible to distinguish from each other by motion correction software (Fig. 2). A careful setup of the subject in the magnet (padding foams) allows for a reduction of these artifacts. External devices, such as a bite bar setup, can be used to further minimize head motion but are rarely used in clinical practice. A careful explanation of the task to the subject may also help to reduce head motion. Head motion should be corrected using currently available methods. Furthermore, nowadays real-time motion correction software is also available.
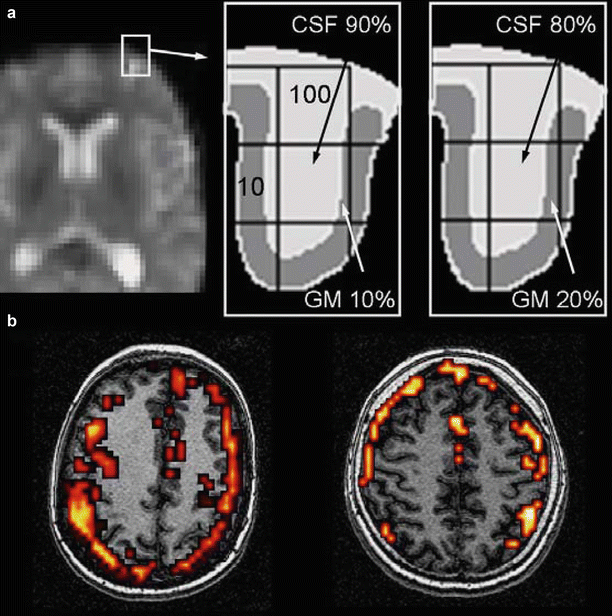
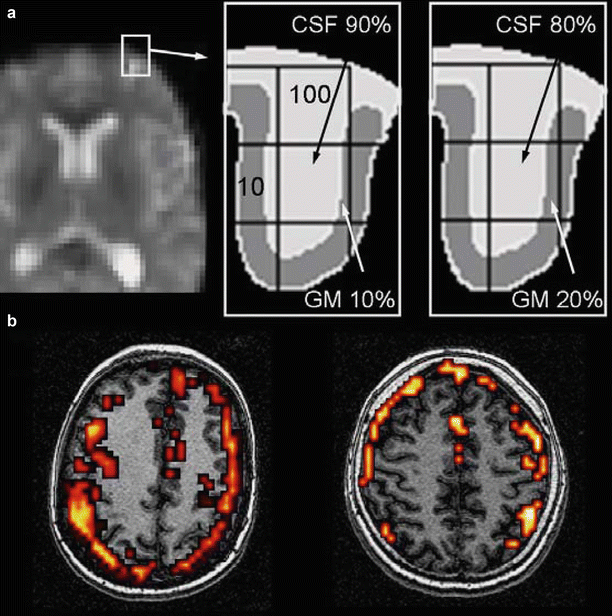
Fig. 2
Head motion artifact. When temporally correlated with task performance, a movement of one tenth of a voxel (300–400 μm) may result in signal changes similar or even larger than the actual signal increase due to task performance which are virtually impossible to distinguish from each other by the motion correction software. Artifacts predominate in regions of tissue interface where changes in signal intensity are high. An example is provided in (a). The diagrams represent an enlargement of a frontal sulcus from the left image. In condition 1, the 4 mm voxel of interest in the middle of the image contains 90 % cerebrospinal fluid (CSF, signal = 100 arbitrary units) and 10 % gray matter (GM, signal = 10) resulting in an average signal of 91 (left diagram). In condition 2, a 0.4 mm motion (one tenth of the voxel size) will result in an average signal intensity of 82 (80 % CSF and 20 % GM, right diagram). (b) Typical head motion artifacts in two different subjects
Cardiac and respiratory motion results in physiological fluctuations of the BOLD signal. Brain motion due to cardiac pulsation is negligible in the motor cortex but becomes significant in the upper brain stem and diencephalon (Enzmann and Pelc 1992). Brain motion is of small amplitude (0.16 mm) but can represent as much as 30 % of the voxel size at ultrahigh spatial resolution (0.5 mm in plane). EPI images are particularly sensitive to changes in resonance frequency which can arise from respiration and are more significant at high magnetic field strengths (Van de Moortele et al. 2002). Noise generated from respiration-induced phase and frequency fluctuations as well as from cardiac-induced fluctuations can be corrected using different algorithms (Pfeuffer et al. 2002), some of them based on physiological recordings (Hu et al. 1995).
1.2.2 Magnetic Susceptibility Artifacts
These artifacts are due to differences in magnetic susceptibility between bone, air, and brain structures. These susceptibility differences generate field gradients which result in an inhomogeneous static magnetic field. The consequence is a signal loss, as well as distortion in EPI images and a blurring in spiral images. These artifacts are particularly predominant in regions containing air and bone, such as sinuses and inner ear structures. They are observed in calcified structures and after brain hemorrhage because of metallic particle deposition, such as in vascular malformations (Fig. 3). They are also frequently observed following brain surgery because of the presence of metallic particles deposited during surgery.
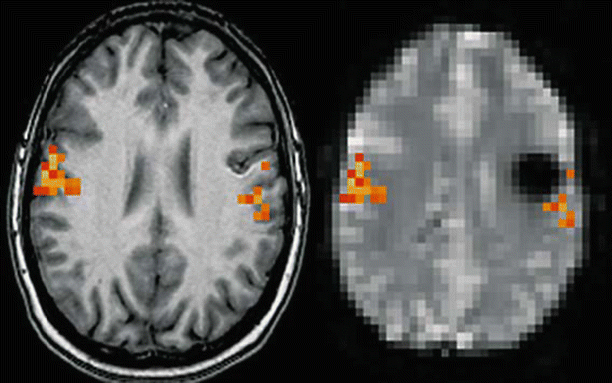
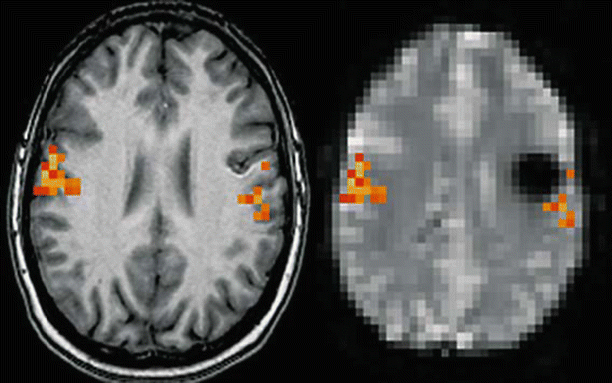
Fig. 3
Magnetic susceptibility artifact. Patient presenting a cavernous angioma of the left central area. Left: Activation map during lip movements superimposed on transverse T1-weighted images suggesting that the lesion is located at a distance from the activation in the primary motor area. Right: Same activation map superimposed on a transverse EPI T2* image showing the lesion surrounded by a large area of signal drop due to T2* shortening susceptibility artifact. Although no activation is visible inside the area of signal drop, it is not possible to conclude that the functional area does not extend inside this area
Magnetic susceptibility artifacts increase with field strength. Their impact can be reduced using higher bandwidth, shorter TE, thinner slices, and better shimming procedures.
1.2.3 BOLD Response Alterations Induced by the Lesion
Neurovascular coupling that underlies BOLD contrast can be altered in normal aging as well as in vascular diseases (Pineiro et al. 2002; D’Esposito et al. 2003; Hamzei et al. 2003). Pathologies that affect neurovascular coupling can affect the characteristics of the BOLD signal. This is particularly important in BOLD fMRI studies in patients with brain tumors because the abnormal vasculature is an essential feature of tumor growth (Folkman et al. 1989). Decreased activation has been reported on the side of the tumor predominantly in high-grade glioma (Holodny et al. 1999, 2000). It has been suggested that negative BOLD responses correlate with decreased neuronal activity (Shmuel et al. 2006) or neuronal inhibition in epilepsy (Kobayashi et al. 2005), but the different factors contributing to decreased BOLD activation in patients with brain tumors are not fully understood. They may also include a loss of autoregulation in the tumor vasculature and venous or pressure effects (Holodny et al. 1999, 2000). Several studies have suggested that regional cerebral blood volume (rCBV) using perfusion MRI is altered in gliomas (Ludemann et al. 2001; Cha et al. 2003; Law et al. 2004) and that fMRI activations are decreased in the vicinity of brain tumors (Holodny et al. 2000; Fujiwara et al. 2004; Hou et al. 2006; Jiang et al. 2010).
In patients with brain arteriovenous malformations (AVMs), flow abnormalities may interfere with fMRI detection of language-related areas (Lehericy et al. 2002). In patients with severe flow abnormalities, results of Wada examination and/or postembolization fMRI have shown that abnormal language lateralization is at least partly due to severe flow abnormalities that impair the detection of the BOLD signal (Lehericy et al. 2002). Neurovascular coupling can be altered in several ways in patients with brain AVM. Hypotension or the presence of a so-called steal phenomenon may negatively influence the normal functions in areas adjacent to the lesion (Barnett et al. 1987; Fogarty-Mack et al. 1996). Changes in cerebral blood flow (Barnett et al. 1987; Young et al. 1990), perfusion pressure (Hassler and Steinmetz 1987; Fogarty-Mack et al. 1996), oxygen metabolism (Fink 1992), autoregulation processes, and vasoreactivity (Barnett et al. 1987; Hassler and Steinmetz 1987; Young et al. 1994; Fogarty-Mack et al. 1996), all of which have been reported in areas adjacent to AVMs, may alter the BOLD signal intensity and detection.
1.2.4 Alternatives to Task-Related fMRI
Preoperative task-related fMRI depends on subject cooperation. In patients with deficits, fMRI may be negative. The choice of tasks is also an important factor to reliably activate eloquent brain areas. These difficulties can be overcome by using various approaches, e.g., by passive movements for motor mapping (Ogg et al. 2009). More recently, new approaches relying on functional connectivity techniques have been proposed. Functional connectivity refers to the temporal correlation between various fMRI signals in spatially remote regions. Functional connectivity can be studied by measuring coherent signal fluctuations in BOLD fMRI time series in the resting brain (Biswal et al. 1995). For instance, resting-state functional imaging studies have thus revealed covariation of these fluctuations in distributed brain networks.
Previous studies have shown that brain tumors induce a loss of functional connectivity (Bartolomei et al. 2006; Guggisberg et al. 2008). Decreased resting-state functional connectivity in the tumor area has been shown to be strongly associated with the absence of eloquent cortex using DES (Martino et al. 2011). In addition, resection of areas with reduced connectivity has been reported to be associated with a low risk of postoperative deficits (Guggisberg et al. 2008). Resting-state fMRI was also efficient to detect the motor and language network when compared with DES (Mitchell et al. 2013). Functional connectivity techniques have therefore a potential to analyze patients with brain lesions noninvasively.
2 Validation of Presurgical Mapping Using Established Reference Procedures
2.1 Direct Electrical Stimulations
Currently, invasive electrophysiological investigations remain the reference procedure (gold standard) for brain surgery, in particular for tumors located near or within eloquent cortical and/or subcortical structures (Keles and Berger 2004; Duffau et al. 2005). Direct electrical stimulations (DES) allow mapping of a large number of motor, somatosensory, and cognitive functions. DES also permits the study of anatomo-functional connectivity by directly stimulating white matter tracts (Duffau et al. 2002, 2003b; Keles et al. 2004). Therefore, DES represent an accurate and reliable technique to localize cortical and subcortical regions at risk regarding brain function. Consequently, a reproducible functional disturbance induced by DES will indicate where to stop with the resection, both for cortical and subcortical structures. Tumor removal is hence performed according to functional boundaries in order to optimize the accuracy of tumor removal in terms of minimizing the risk of postoperative functional deficits.
DES have some limitations, however. They only allow loco-regional and not whole brain mapping. Further, they are time-consuming, and the number of tasks that can be performed during surgery is limited. Therefore, DES should still be combined with other metabolic and functional methods.
2.1.1 Basic Principles
Electrical stimulations increase membrane excitability, via an initial phase of passive modification of the local membrane potential (MP) at the level of the cathode (the negative electrode). The inner side of the membrane becomes progressively less negative than the outer side (the membrane becomes inversely hyperpolarized with regard to the anode). The intensity of this phenomenon depends on the parameters of electrical stimulation and the characteristics of the membrane (Jayakar 1993). Stimulation of the membrane is easier at the level of the initial segment of the axon in myelinated fibers and in fibers of greater diameter (Ranck 1975). If the MP reaches the depolarization threshold, voltage-dependent ion channels will open and allow the entry of Na+ ions inside the neuron. This Na+ entry will invert the MP between +20 mV and +30 mV. A secondary output of K+ ions, associated with an inhibition of the inward flux of Na+ ions, brings the MP back to its resting state. Once generated, this rapid sequence of MP fluctuations – the action potential – is always the same, regardless of the stimulation parameters (law of all or nothing).
2.1.2 Risks Associated with DES
Direct electrical stimulations can damage the brain. Tissue damage can result from numerous causes. Accumulation of a negative charge at the level of the cathode or through the production of metal ions at the level of the anode can damage the brain (Agnew and McCreery 1987). This can be prevented by biphasic impulses because the second stimulus inverses the effects of the first one. Excessive heat produced by hydrolysis induces vacuolization and chromatolysis (Doty and Barlett 1981). A leaking of the intracellular current, which goes from the anode to the cathode through the cytoplasm, can damage the mitochondria and the endoplasmic reticulum (Pudenz et al. 1977). Repetitive and synchronous stimulations of neurons can alter neuronal homeostasis (Fertziger and Ranck 1970; Agnew and McCreery 1987). These risks are directly linked to the density of the charge. Animal studies have shown that, when the charge does not exceed 55 μC/cm2/phase, lesions do not occur (Gordon et al. 1990). Stimulations can also generate seizures. The frequency of seizures is estimated at 5–20 % (Sartorius and Berger 1998). The monitoring of the stimulation using intra-stimulation electrocorticographic recording avoids the occurrence of afterdischarge (Lesser et al. 1984), except in children who have nonmyelinated fibers (Jayakar et al. 1992). Recording electrodes should ideally be located in the immediate proximity of each stimulation and therefore should follow the stimulation electrode. They should also have a small diameter to allow stimulation of the cortex.
Finally, the accumulation of any conductive substance such as cerebrospinal fluid or blood can favor the distribution of current beyond the target tissue, thus increasing the risk of false negatives: Any stimulated structure must therefore be kept dry.
2.1.3 Practical Stimulation Methods
The optimal stimulation parameters (best benefit/risk ratio) have been extensively studied (Nathan et al. 1993; Duffau 2004). They can be significantly modified by the degree of cerebral maturation and fiber myelination (Jayakar et al. 1992), as well as by anesthetic drugs and pathological processes (tumor, epilepsy, postictal status) (Jayakar 1993).
Bipolar stimulation is usually performed using a probe with two tips separated by 5 mm (Fig. 4) and the following parameters: rectangular impulses, biphasic current at 50 Hz (Lesser et al. 1987) or 60 Hz (Ojemann et al. 1989; Berger 1995), and intensities of 1–18 mA. Under local anesthesia, current intensities of less than 6–8 mA with an impulse duration of 0.3 ms (Lesser et al. 1987) to 1 ms (Ojemann et al. 1989; Berger 1995) are standard parameters. Typically, the stimulation intensity is progressively increased from 4 mA (under general anesthesia) or 1 mA (under local anesthesia), by increments of 1 mA in order to find the optimal threshold generating responses without causing seizures.
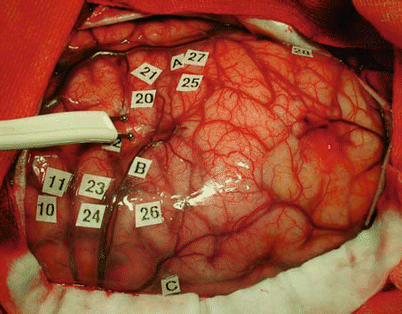
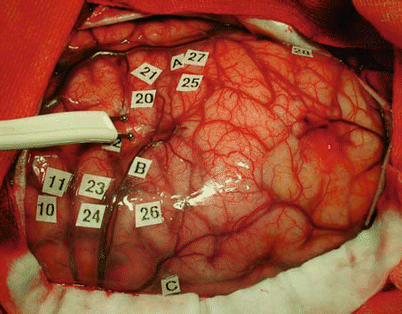
Fig. 4
Intraoperative view of a patient showing bipolar stimulation using a probe with two tips separated by 5 mm. During surgery, the probe is placed on the cortical surface of the patient. Stimulation sites are labeled using small tags
In children, the rates of response are lower than in adults (less than 20 % <5 years or even no response <1 year) because of the immaturity of fibers (higher chronaxie in nonmyelinized fibers). Therefore, a progressively and alternately sequential increase of impulse intensity and duration has been proposed (Jayakar et al. 1992).
2.1.4 Neuropsychological Evaluation
Defining the eloquent nature of cortical areas using DES requires appropriate tasks and an accurate recording of the clinical responses to determine whether there is any interference with function during stimulation of the site. For this purpose, speech therapists or psychologists are present in the operative room in order to interpret the disorders induced by DES (Duffau et al. 2002).
Mapping of motor functions can be performed in awake patients or under general anesthesia, by inducing involuntary motor responses. Awake patients are generally passive during stimulation. Rarely, when looking for negative motor areas in the premotor cortex (Luders et al. 1995), the patient is asked to make regular movements during the stimulation. Changes in movement parameters are recorded (slowdown, decrease in precision or amplitude, interruption). Under general anesthesia, small movements can be overlooked. This is a frequent problem for neck movements because the intubation cannula often prevents muscular contractions from being perceived. Concomitant intraoperative electromyographic recording and motor evoked potentials improve the detection of positive responses (Yingling et al. 1999). These methods are constraining, however. They require additional equipment, the presence of an electrophysiologist in the operating room, and the positioning of electrodes on the entire hemibody contralateral to the stimulation side if the lesion is in the vicinity of the corona radiata and/or the internal capsule (Duffau et al. 2003a). Sensory functions are mapped by eliciting dysesthesia described by the patient himself intraoperatively (responses are therefore subjective).
For cognitive function, the study of language (spontaneous speech, naming, comprehension, etc.), calculation, memory, reading, or writing evaluation is performed in awake patients by generating transient disturbances (Ojemann et al. 1989; Duffau et al. 2002). The choice of the most appropriate tests is critical. Eloquent sites can be detected only if the proper function has been tested. This is why sensitive tasks are used rather than specific ones.
Language mapping usually includes a counting test followed by an object-naming task. The counting test is likely to alter articulatory production, while the following automatic series are being carried out (slowing down, dysarthria, anarthria, complete speech arrest, more or less associated with facial movements and/or hoarseness and/or automatic swallowing). The object-naming task induces various symptoms such as articulatory disorders, pure anomia, phonemic or semantic paraphrasias, or even perseverations. These tasks are short (less than 4 s duration) and therefore compatible with surgical requirements under local anesthesia). Depending on the localization of the lesion, a third more specific task is also performed. This task is chosen in each patient based on the individual cortical language organization, as evaluated preoperatively by a neuropsychological assessment and functional neuroimaging. Tasks include verb generation, tasks in foreign languages in bilingual individuals, memory, calculation, repetition, reading, or even comprehension tasks for posterior temporoparietal lesions (Gatignol et al. 2004).
2.1.5 Coregistration with Functional Imaging Data
Comparison between DES and functional imaging data has been performed using several methods. Initially, simple visual comparisons were obtained between intraoperative views and fMRI data (Jack et al. 1994; Yousry et al. 1995). Coregistration has also been performed by comparing pictures of DES positive sites with the preoperative 3D rendering of the brain (Pujol et al. 1996; Lehericy et al. 2000b) (Fig. 5). These methods have limitations, however, as they are not quantitative. 3D rendering displays brain activation as viewed transparently from the surface of the brain: Therefore, the display includes activation that is located in the depth of the sulci and gyri. More recently, coregistration has been obtained using stereotactic neuronavigation procedures (Lehericy et al. 2000b; Krings et al. 2001b; Krishnan et al. 2004). This technique allows a 3D multiplanar comparison of the DES positive sites with functional data, as well as measurements of distances and overlap.
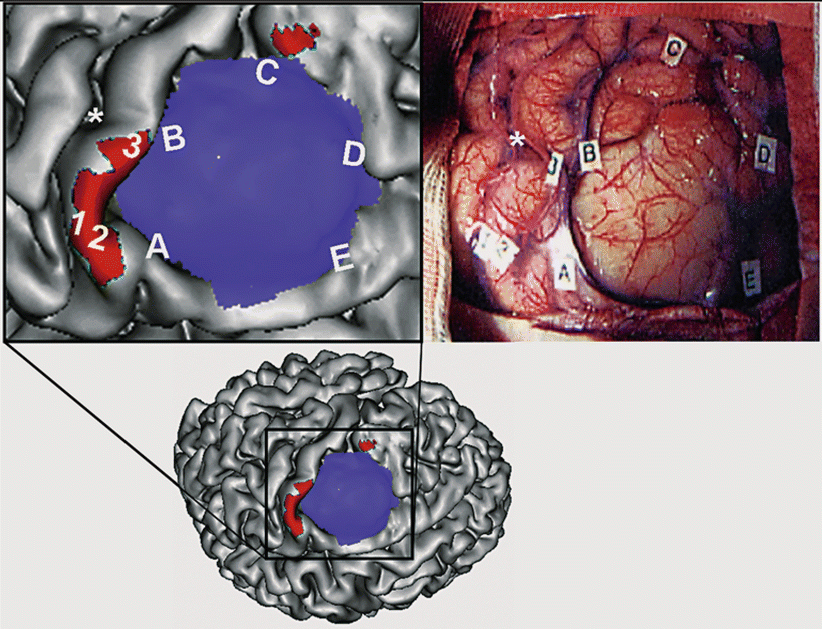
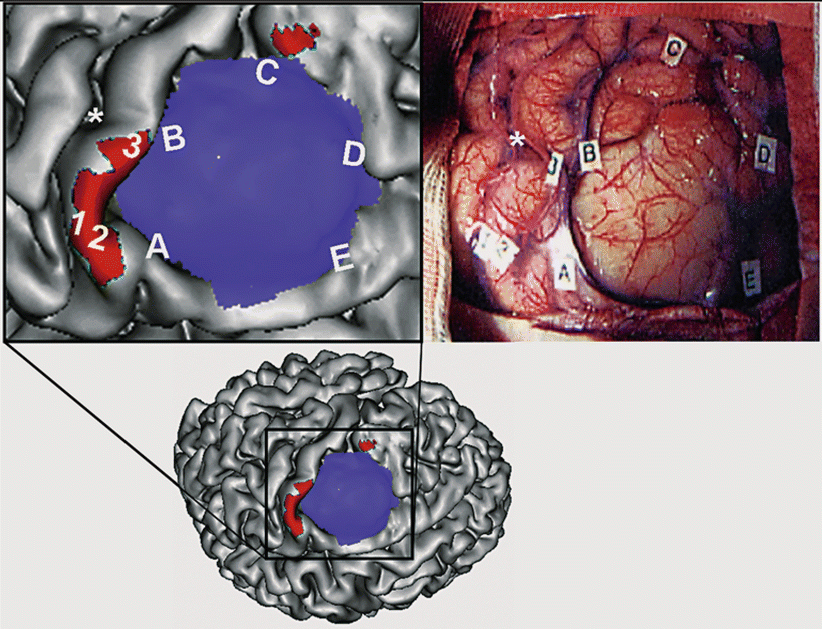
Fig. 5
Correspondence between fMRI and intraoperative cortical stimulation. Upper left: Surface rendered of the cortex in a patient with a left premotor low-grade glioma (enlarged view of the figure in the lower row). The tumor is represented in blue. Activation obtained during performance of right-hand movement is shown in red. Upper right: Intraoperative view of the same patient (same orientation). The letters outline the tumor margins. The numbers indicate the stimulation sites that elicited hand movements. Note the good correspondence between the fMRI activation map and direct electrical stimulations (Color figure online)
2.1.6 Limitations of Functional and DES Comparison
DES and functional imaging methods provide fundamentally different types of data. DES interfere directly by blocking the ability of the patient to perform a task. Therefore, it is assumed that DES depict critical language areas only. In contrast, functional imaging methods provide activation maps obtained during performance of motor, language, or cognitive tasks. Consequently, imaging techniques do usually not only include critical areas but also activity in nonessential areas that are only associated with task performance but could be resected. Functional maps are displayed using a statistical threshold. Therefore, a low threshold may increase both the number of nonessential areas and the size of the critical areas, whereas a stringent threshold may not allow critical areas to reach statistical significance. Craniotomy and debulking may induce deformation, which impairs image registration (Hill et al. 2000; Krings et al. 2001b). Finally, tasks performed during neurofunctional imaging and intraoperative procedures are not identical because of the different setups (Lurito et al. 2000). These issues need to be kept in mind when comparisons between the different techniques are performed.
2.2 Intracarotid Amobarbital Procedure (IAP) or Wada Test
The intracarotid amobarbital procedure (IAP), or Wada test, was first reported as a means to identify the hemisphere of language dominance in patients with epilepsy (Wada and Rasmussen 1960). IAP was then modified to measure memory function in patients undergoing surgery for epilepsy (Milner et al. 1962). The role of IAP is to assess language and amnesic risks by ensuring that the hemisphere contralateral to the operated one is able to subserve language and memory functions. Thus, IAP helps to predict and prevent postoperative language and memory deficits and in the end to give an estimate of linguistic and neuropsychological outcome (Sperling et al. 1994; Loring et al. 1995).
2.2.1 Methods
IAP is performed in the angiography suite in the presence of a neurological and neuroscience team (Rausch et al. 1993; Akanuma et al. 2003). The patient is positioned on the angiography table. EEG monitoring is performed. After local skin anesthesia and femoral artery access, a diagnostic catheter is placed selectively in the internal carotid arteries (ICA) under fluoroscopic guidance. Then amobarbital is injected in the ICA for either side separately. Therefore, the IAP induces a temporary inactivation of the cortex supplied by the anterior and middle cerebral arteries in the hemisphere ipsilateral to the injection. Rarely, the two hemispheres are tested on two consecutive days. During the 10–15 min of the procedure, patients receive a battery of language and memory tasks following amobarbital injection (Jones-Gotman 1987). Language tasks usually include speech production (serial speech, naming), speech reception (simple motor commands, token test), and other speech tasks, such as reading aloud and spelling. Tasks are scored so that a lateralization index (LI) can be calculated for each task. The LI can be expressed as the difference between the scores during left injection minus the scores during right injection of amobarbital, divided by the maximum possible score.
2.2.2 Risks and Limitations of the Wada Test
The IAP has several disadvantages, however (Simkins-Bullock 2000). Risks include those of intracarotid catheterization. Following cerebral angiography, the ischemic event rate has been estimated between 0.3 and 1.8 % (0.07–0.3 % permanent) (Dion et al. 1987; Cloft et al. 1999). Test–retest reliability and external validation cannot be performed. IAP evaluation is performed within a short period of time (approximately 3–10 min). Anesthesia can induce behavioral effects (e.g., aphasia, attention deficits, neglect, and somnolence). Amobarbital injected via the ICA will inactivate the amygdala and the anterior hippocampus but never or rarely the posterior two thirds of the hippocampus (Jack et al. 1989; Hong et al. 2000b). This represents a critical concern for the validity of memory assessment. Moreover, in some patients, mesial temporal perfusion can be unaffected during IAP (de Silva et al. 1999). In addition, IAP also inactivates the rest of the hemisphere ipsilateral to the injection and in some patients also the contralateral hemisphere (Hong et al. 2000b). To overcome these limitations, selective IAP via an injection in the posterior cerebral artery has therefore been proposed to test for memory function (Jack et al. 1989). The risk of morbidity of this procedure is much greater than via direct ICA injection, and thus it is not widely used (Jack et al. 1989). For language functions, the IAP provides information on the hemispheric language dominance but not on the anatomical location of language areas and their relation to the lesion. Lastly, the total direct costs of the Wada test has been estimated 3.7 times higher than that of fMRI (Medina et al. 2004).
2.2.3 Validation of the Wada Test
Validation of IAP has been performed in several ways. Recording of intrahippocampal activity using depth electrodes has shown that the EEG background activity can be significantly suppressed in the posterior hippocampal regions even in the absence of amobarbital perfusion in these areas (Urbach et al. 1999). Using HMPAO-SPECT, hypoperfusion in the territories of the anterior and middle cerebral arteries was observed during IAP (de Silva et al. 1999). Hypoperfusion in medial temporal structures was noticed in the great majority of these patients (de Silva et al. 1999). Further, in the epileptogenic hemisphere, the degree of hippocampal damage correlated with the impairment in Wada memory performance (Sass et al. 1991; O’Rourke et al. 1993; Davies et al. 1996).
In a few patients with unilateral temporal lobe epilepsy, IAP may also paradoxically lateralize memory function. In these patients, IAP showed poorer memory performance in the non-epileptogenic hemisphere (Davies et al. 1996; Rouleau et al. 1997; Detre et al. 1998; Spencer et al. 2000). Paradoxical IAP memory lateralization (i.e., ipsilateral to the seizure focus) was still concordant with fMRI lateralization scores in two patients with temporal lobe epilepsy (Detre et al. 1998). Spencer et al. (2000) suggested that in patients with medial temporal lobe epilepsy not well lateralized by noninvasive evaluation and in patients with neocortical or mesial frontal epilepsy, IAP may provide incorrect localization which ultimately alters surgical management (Spencer et al. 2000). This paradoxical lateralization has rarely been reported using FDG-PET (Sperling et al. 1995; Nagarajan et al. 1996). In such cases, combined FDG-PET with IAP studies did not report memory impairment contralateral to the hypometabolic zone (Salanova et al. 1992).
3 Important Results of Validation Studies in Brain Tumors and Epilepsies, Overview, and Current State
3.1 Motor Function
3.1.1 Functional Mapping
Validation of more recent functional noninvasive methods, such as fMRI, magnetoencephalography (MEG), and transcranial magnetic stimulation (TMS), can be performed by comparison with earlier techniques such as PET. Nevertheless, DES remain the current reference procedure, and therefore comparison of functional noninvasive methods with DES is the best method of validation.
Functional mapping of motor areas can be performed either to localize the central area or to locate motor areas at risk of functional deficits. Therefore, there are two different levels of validation.
The first level is to determine whether functional imaging methods can accurately localize the central sulcus. In the normal brain, the hand area and therefore the central sulcus can be accurately localized using anatomical landmarks only (Yousry et al. 1997) (Fig. 6). In patients with brain tumors, however, mass effect frequently distorts normal cortical anatomy and can make localization of the central area difficult by using anatomical landmarks alone (Lehericy et al. 2000b). In such cases, preoperative functional localization is of special interest. See also chapter “Task-based presurgical functional MRI in patients with brain tumors”.
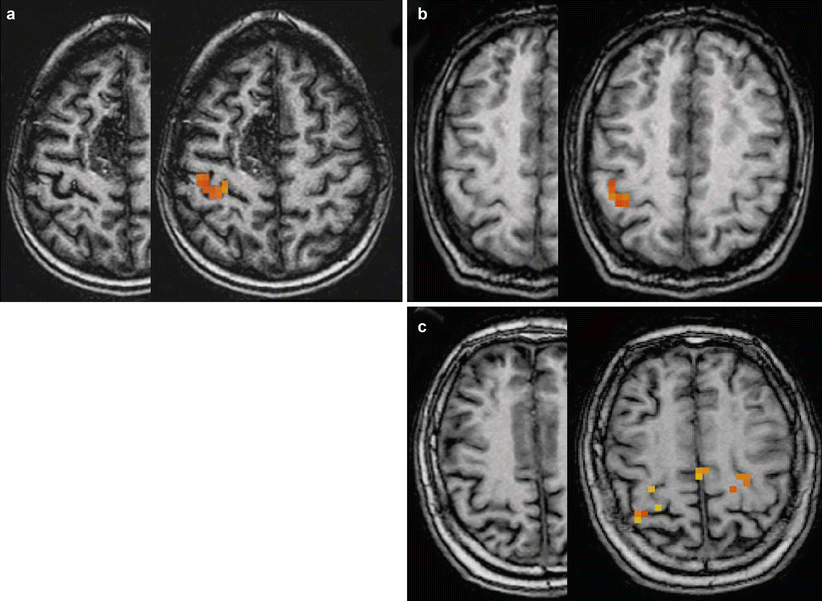
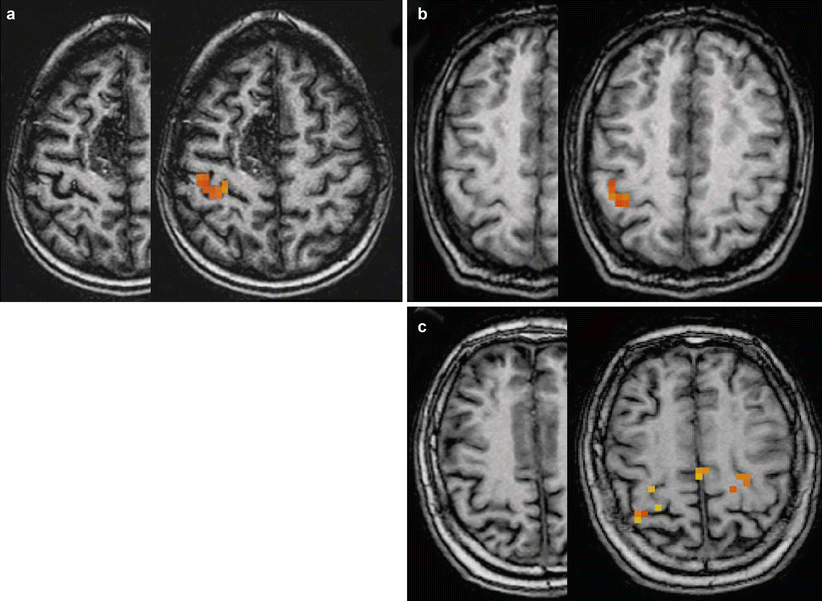
Fig. 6
Typical variations of anatomy of the hand area. Movements of the left hand in three different subjects. Activated pixels are overlaid onto anatomical T1-weighted images in the right hemisphere. (a) Typical omega-shaped hand area (patient with a right medial frontal arteriovenous malformation). (b) Epsilon-shaped hand knob. (c) More complex aspect of the hand area (three-digit aspect)
fMRI vs. PET
Compared with PET, 96 % of the fMRI activation peaks were located on either the same or adjacent sulci and gyri (Bittar et al. 1999a).
fMRI vs. DES
Compared with DES, fMRI was very reliable in localizing the motor cortex, with complete or almost complete agreement using visual comparison with 3D MRI (Jack et al. 1994; Yousry et al. 1995; Pujol et al. 1996, 1998; Roux et al. 1999) (Fig. 5) or preoperative ultrasonography (Fandino et al. 1999), coregistration of preoperative pictures with 3D rendering of the brain (Puce et al. 1995; Lehericy et al. 2000b), or neuronavigation procedures (Hirsch et al. 2000; Lehericy et al. 2000b). Discordant results were mostly observed when no correlation was possible (no activation or movement artifacts on fMRI, no response using DES). 15H2O PET was also reliable in showing the central area compared to DES (Reinges et al. 2004).
fMRI vs. TMS
Compared with TMS, fMRI peaks produced no motor evoked potential (MEP) when located more than 2 cm away from TMS sites (Krings et al. 1997b).
The second level is to determine whether functional imaging data can accurately localize motor areas and to assess how reliable this information is to assess where to stop with the surgical resection in order to prevent functional deficits.
fMRI vs. PET
Compared to PET, fMRI yielded very similar results (Bittar et al. 1999a). The average distance between fMRI and PET activation peaks was 7.9 ± 4.8 mm, with 96 % of the peaks being located on either the same or adjacent sulci or gyri. Overlapping of voxels activated by each modality occurred in 92 % of the studies.
Agreement between PET and fMRI functional localization was 95.6 % for intra-axial lesions and spatially concordant as determined by intracranial stimulation (Bittar et al. 1999b). PET results were overlapping with DES in 60–92 % (<1 cm) and neighboring in 29–75 % (<2 cm) (Krings et al. 2001b; Reinges et al. 2004). The mean localization difference between fMRI and PET was 8.1 ± 4.6 mm (range, 2–18 mm) (Reinges et al. 2004). Another study reported that the evaluation of PET findings by cortical stimulation yielded a 94 % sensitivity and a 95 % specificity for the identification of motor-associated brain areas (Schreckenberger et al. 2001). PET results for extra-axial lesions were even superior to those obtained with fMRI with 75 % overlapping and 25 % neighboring activation, showing no contradictory results (Reinges et al. 2004).
fMRI vs. DES
Several studies have compared the accuracy of fMRI localization according to DES. Early studies in 28 patients with surgical brain lesions reported that 100 % of positive MR activation sites were within 20 mm of DES positive sites and that 87 % of correlations were within 10 mm of DES (Yetkin et al. 1997). Another study in 8 patients with brain tumors reported a good correlation between fMRI activation and DES sites, with all fMRI activations related to positive DES responses (Roux et al. 1999). In patients with intra-axial lesions, overlapping results were obtained in 25–63 % (<1 cm) and neighboring in 29–75 % (<2 cm), while contradictory results were observed (Krings et al. 2001b; Reinges et al. 2004). Results are less good in patients with extra-axial lesions, with contradictory results in 42 % (activation in a different gyrus or >2 cm from DES site) (Reinges et al. 2004). In another study, quantitative comparison of noninvasive methods with DES did not reveal a significant difference in accuracy (Towle et al. 2003). Other more studies reported an agreement between fMRI and DES regarding localization in 92 % (Lehericy et al. 2000b), 92.3 % (Spena et al. 2010), 83.7 % (Gonzalez-Darder et al. 2010), and 77 % of subjects (Bartos et al. 2009). The choice of statistical thresholds to display activation maps is also an important factor to take into account as the optimal threshold varies between subjects (Chang et al. 2010).
TMS vs. DES/fMRI
TMS combined with neuronavigation has also been used for presurgical motor mapping (Picht et al. 2011; Coburger et al. 2013; Krieg et al. 2013) evaluated by comparison to fMRI and DES. TMS sites were close (within 5 mm) to DES positive sites (Picht et al. 2011). Compared to DES, TMS responses fell within 1 cm of the electrical cortical stimulation sites (Krings et al. 1997a; Forster et al. 2011).
TMS sites further than 2 cm away from fMRI peaks produced no motor evoked potentials (MEPs) (Krings et al. 1997b, 2001b), while the mean distance between the fMRI and TMS activation peaks was below 1.5 cm (Krings et al. 2001a; Forster et al. 2011). Further, TMS was able to locate the motor cortex when fMRI failed (Coburger et al. 2013).
FMRI vs. MSI
Magnetic source imaging (MSI) using somatosensory stimulation has also been evaluated in patients with brain tumors (Schiffbauer et al. 2002). In one study, the distance between two corresponding points determined using MSI and DES was 12.5 ± 1.3 mm for somatosensory–somatosensory and 19 ± 1.3 mm for somatosensory–motor comparisons. Intraoperative sites at which DES evoked the same patient response exhibited a spatial variation of 10.7 ± 0.7 mm (Schiffbauer et al. 2002).
Overall, these studies suggest that the localization accuracy among the different functional methods is similar. Earlier studies have suggested that in patients with a minimum distance of 2 cm between tumor and activation site, no deficits in motor function are expected after surgery (Mueller et al. 1996; Yetkin et al. 1997). Other studies have suggested that even a shorter distance of <1 cm (lesion to function site) can be achieved (Krishnan et al. 2004; Bartos et al. 2009). Therefore, fMRI methods are considered a useful adjunct to DES.
The value of functional imaging techniques for the accurate localization of functionally important areas at risk for postoperative deficits can also be assessed empirically by a comparison to the postoperative outcome. In this regard, fMRI has proven to be very accurate for the prediction of occurrence of motor and language deficits after medial frontal lobe surgery (Krainik et al. 2001, 2003; Wood et al. 2011).
It should be kept in mind that fMRI results should be interpreted cautiously as they highly depend on several factors, such as the quality of the examination (movement artifacts), data processing (smoothing), statistical thresholds, and ultimately the experience of the reader. Lastly, functional brain mapping techniques alone cannot assess subcortical white matter tracts. Therefore, combined fMRI and DTI fiber tracking methods have the potential to provide a more complete presurgical mapping than any other functional technique by its own.
3.1.2 DTI Fiber Tracking of the Corticospinal Tract
Compared to the already described functional imaging techniques, fewer validation studies have been published for DTI fiber tracking. Previous studies have shown the reconstruction of well-known fiber tracts, including the corticospinal, long association (Roux et al. 1999; Mori et al. 2002), and brain stem fiber tracts (Stieltjes et al. 2001). Results of fiber tracking have also been correlated with postmortem animal studies in formalin-fixed hearts in rabbits (Holmes et al. 2000) and in the skeletal muscle of rats in vivo (Damon et al. 2002). In vivo DTI has been compared to ex vivo DTI and also to the uptake transport of wheat germ agglutinin–horseradish peroxidase (WGA-HRP)-stained histological sections in the macaque monkey (Dauguet et al. 2007). A good correspondence has been reported between DTI fiber tracts originating in the left somatosensory cortex and the histological reference. In humans, tractography results have been compared with the expected known anatomy, dissections, or DES (Lawes and Clark 2010).
Validation studies of DTI fiber tracking have been performed using subcortical DES in patients with brain lesions. Two studies from the same group used cortical positive DES as seeding points for fiber tracking (Berman et al. 2004; Henry et al. 2004). Tracts originating from the mouth motor positive DES matched the known connections of the primary motor area of the face. For the corticospinal tract, a good agreement was reported in 92–95 % between DTI reconstructed tracts and positive functional subcortical DES sites (Coenen et al. 2003; Kamada et al. 2005; Bello et al. 2008; Ohue et al. 2012; Zhu et al. 2012). The mean distance range between the stimulated sites and the corticospinal tract was 6–14 mm (Bozzao et al. 2010; Gonzalez-Darder et al. 2010; Prabhu et al. 2011; Zhu et al. 2012; Vassal et al. 2013). These distances depended on the intensity used for stimulation (Kamada et al. 2009). DTI tractography may underestimate the presence of functional fibers in the border of the lesions (Spena et al. 2010). Finally, the topography of the tract, i.e., inside or at the boundary of the tumor, has been confirmed by DES (Bello et al. 2008, 2010).
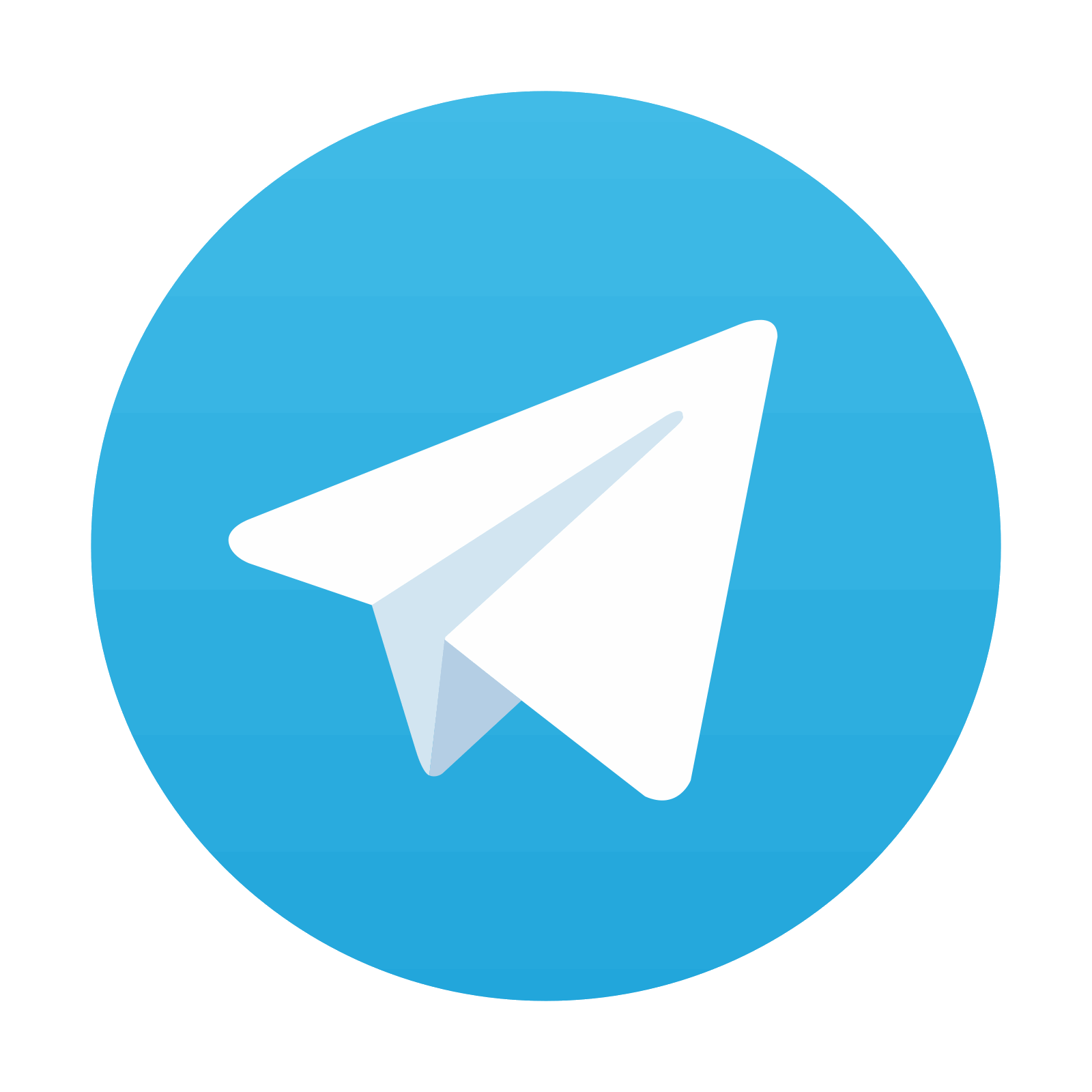
Stay updated, free articles. Join our Telegram channel
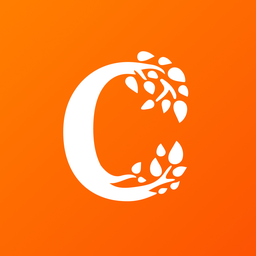
Full access? Get Clinical Tree
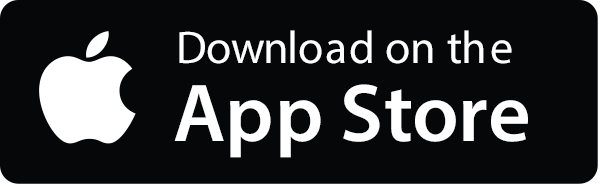
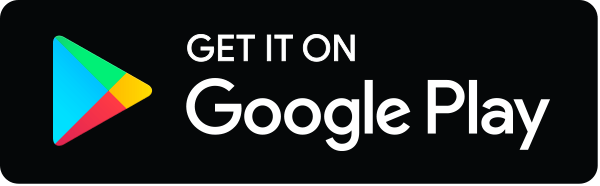