Magnetic resonance image-guided focused ultrasound surgery (MRgFUS) has surfaced as a viable noninvasive image-guided therapeutic method that integrates focused ultrasound (FUS), the therapeutic component, with magnetic resonance imaging (MRI), the image guidance module, into a real-time therapy delivery system with closed-loop control of energy delivery. The main applications for MRgFUS of the brain are thermal ablations for brain tumors and functional neurosurgery, and nonthermal, nonablative uses for disruption of the blood brain barrier (BBB) or blood clot and hematoma dissolution by liquification. The disruption of the BBB by FUS can be used for targeted delivery of chemotherapy and other therapeutic agents. MRI is used preoperatively for target definition and treatment planning, intraoperatively for procedure monitoring and control, and postoperatively for validating treatment success. Although challenges still remain, this integrated noninvasive therapy delivery system is anticipated to change current treatment paradigms in neurosurgery and the clinical neurosciences.
Magnetic resonance image-guided focused ultrasound surgery (MRgFUS) of the brain is expected to revolutionize central nervous system (CNS) therapy and, in general, to change current treatment paradigms. The potential clinical applications include but are not limited to the treatment of benign and malignant brain tumors, vascular malformations, and ischemic or hemorrhagic stroke, as well as the nonablative targeted delivery of therapeutic drugs, genes, and antibodies. The integration of magnetic resonance imaging (MRI) guidance with advanced phased-array ultrasound technology has allowed this new therapeutic modality to emerge as a viable noninvasive alternative to current invasive and minimally invasive treatments. The development of MRgFUS therapy delivery systems with large numbers of phased-array transducer elements has permitted complete, noninvasive transcranial delivery of ultrasound waves through the intact bony skull, termed transcranial MRgFUS (TcMRgFUS).
Multiple recent articles elaborate on MRgFUS’ historical progression by detailing the step-wise advancement of focused ultrasound (FUS) technology to MRgFUS’ current state. Since the discovery of the piezoelectric effect (the production of acoustic energy when electric current is applied to a piezoelectric crystals) by Pierre and Jacques Curie in 1880, its subsequent incorporation into acoustic systems in 1918, and the first demonstration of its therapeutic effects in the brain by Lynn and colleagues in 1942, FUS has visibly materialized into the present-day therapeutic instrument. Investigated by Heimburger, FUS for brain tumor thermal ablation began with a single-element FUS transducer without image guidance and required a relatively large craniotomy to fit the transducer. In the early development of FUS, Lars Leksell, the acknowledged pioneer of radiosurgery, became an early advocate of FUS’ role in neurosurgical applications. However, Leksell abandoned FUS as a potential lesioning device because of its need for a craniotomy to create an acoustic window, which made it invasive. He rather focused on targeted ionizing radiation, an effort that resulted in the development of the gamma knife in 1967. The early failure of FUS brain applications was due to the inability to focus through the intact bony skull that necessitated a craniotomy and the lack of an imaging modality to accurately and reliably visualize brain tumors and monitor temperature elevation and tissue changes.
However, these fundamental technical challenges for the most part have been successfully identified and commensurately addressed today. The focusing through the skull has been resolved by correcting phase aberrations caused by unequal bone thickness. The correction method uses skull thickness measurements computed from computed tomography (CT) and entails focusing through a large number of phased arrays that are arranged in a helmet surrounding the skull. The development of MRI in the 1980s and MRI thermometry in the early 1990s were significant in the advancement of MRgFUS. MRI as a noninvasive imaging modality has made possible the essential anatomic and functional roadmap. It unquestionably provides accurate preoperative definition and delineation of the target lesion and preoperative planning ability, intraoperative monitoring of ultrasound-induced thermal changes through magnetic resonance thermometry (temperature-sensitive maps), and postoperative validation of treatment success. In the early 1990s, the collaboration of researchers at the Brigham and Women’s Hospital (BWH) with General Electric (GE Health care, Milwaukee, Wisconsin) and Insightec (Haifa, Israel) resulted in the integration of FUS and MRI into a single image-guided therapy delivery system, MRgFUS. This development marks an important milestone for FUS as a therapeutic instrument for soft tissue tumors in general and intracranial tumors in particular. These developments have allowed FUS to re-emerge as a potential therapeutic instrument for the treatment of brain tumors and other CNS diseases. Therefore, when compared with related interventional procedures, MRgFUS of the brain has indisputably had to overcome disproportionate and challenging obstacles.
Inspired by the results of MRgFUS for the treatment of fibroadenomas, clinical trials were initiated in breast cancer and later for uterine fibroids. That last endeavor of MRgFUS ablation of uterine fibroids subsequently received approval by the US Food and Drug Administration (FDA). Consequently, this technology has been released to the surgical arena for further clinical applications and clinical trials in noncranial diseases such as cancers of the prostate, liver, and bone.
Milestones for transcranial delivery of FUS
In the last decade, a progression has occurred from the use of a single-element FUS transducer with and without MRI guidance that required a craniectomy or craniotomy as an acoustic window to an integrated multielement phased-array ultrasound with MRI guidance. Most recently, however, the completion of a noninvasive TcMRgFUS system demonstrates a positive step toward resolution of an important limitation in transcranial delivery of FUS: the intact bony skull. Unlike air- and gas-containing cavities, the bony skull is not an absolute barrier to the transmission of acoustic waves and acoustic energy deposition. Bone remains a relatively impenetrable acoustic barrier because of its high acoustic attenuation coefficient that proportionally reflects its thickness and density. Therefore, in the absence of an acoustic window provided by a craniotomy, the intact osseous skull creates two major challenges when performing transcranial FUS:
- 1.
Defocusing due to the inhomogenous thickness and density of the skull that results in phase distortions and aberrations that, in turn, inhibit the establishment of a focal point of convergence, the consequence of which is inaccurate targeting and noncontrolled energy deposition
- 2.
Heating of the skull and near-field soft tissues caused by the absorptive properties of bone with a proportional decrease in acoustic energy that reaches the deeply located lesions.
The current phased-array configuration addresses these challenges from defocusing by permitting adjustments of the phases of each element to correct for skull-induced phase aberration. Bone’s high acoustic attenuation coefficient causes scatter and energy absorption. Irregular bone thickness and inhomogeneous density further augment these latter artifacts and cause severe aberrations and phase distortions that result in defocusing. Preprocedural CT scans provide the information necessary to allow phased-array transducers to correct and compensate for inhomogenous skull thickness and density via transcranial focusing and acoustic modeling. With the advent of the first prototype integrated MRgFUS brain system developed by Insightec, overheating of the skull and the near field tissues can be addressed by two methods:
- 1.
A helmet-type, hemispherically configured phased-array transducer that spatially spreads the transducer elements and consequently distributes heating across a larger surface area, while it simultaneously deposits sufficient thermal energy at the focal point of convergence (the target lesion)
- 2.
A cold water cap containing circulating degassed water chilled to about 15°C that is placed between the patient’s head and transducer to provide an active cooling system for the scalp and the skull ( Fig. 1 ).
Fig. 1
Transcranial treatment system (Exablate 4000, Insightec, Haifa, Israel). (A) The helmet-type, hemispherically configured phased array transducer results in spatial spreading of the transducer elements and consequently distributes heating across a larger surface area. (B) This is placed over the patient’s head. A degassed cold-water cap is placed between the patient’s head and the transducer.
Principles of MRgFUS
MRgFUS combines and integrates a therapeutic and imaging modality, the FUS device, and MRI, respectively, into a single image-guided therapy delivery system. FUS, the therapeutic instrument, causes direct ultrasound-induced nonthermal (nonablative) and thermal (ablative) changes in the target tissue. As an ablative modality, FUS’ effects on tissue are caused by thermal heating. As a nonablative tool, FUS can reversibly disrupt the BBB, resulting in an increase in cerebrovascular capillary permeability and a consequent increase in the flux of relatively larger, nonlipophilic, and high molecular weight molecules that otherwise would not cross the BBB. Other nonthermal effects include an increase in cell membrane permeability for use in gene therapy and neuromodulation.
Ultrasound penetrates through tissues without depositing any significant amount of energy. The focusing of ultrasound beams results in a concentration of energy at the focal point. At a given absorption coefficient corresponding to the specific tissue, in thermal ablations, the generation of thermal energy is maximal at the point of convergence where the summation of the acoustic intensity is highest. Overlapping sonications are delivered to the target tissues, generating heat at the focal point that exceeds a 60°C thermal coagulation threshold. FUS induces thermal coagulative necrosis of approximately 1 to 3 mm in diameter (perpendicular to the beam) and about twice as long in length (parallel to the beam). Given the small focal point per sonication, multiple strategies are employed in an effort to increase the focal point size and decrease overall treatment time. Strategies to increase the ablation volume include “cavitation-enhanced ablation” and treating multiple sites simultaneously.
Before delivering the sufficient treatment doses, subthreshold, noncoagulative low-power sonications are delivered to identify the location of the focus and to provide correct targeting of the preselected location or lesion. Magnetic resonance thermometry confirms the focal point on the target lesion by detecting only short and small temperature elevations below 60°C at a time when there are no tissue changes. After this targeting confirmation, high-power focused sonications (500 to 20,000 W/cm 2 ) of short duration (1 to 60 seconds) are administered. Because of the relatively long cooling time (over minutes) between sonications, the overall treatment time is lengthy. Cooling is necessary to avoid the summation of heat effects, which, without cooling, can result in larger tissue volumes than the focal volume. Unlike probe-delivered thermal ablations with wide gradients, FUS has steep thermal gradients. Short sonication times prevent substantial cooling effects from blood flow and perfusion and simultaneously reduce heat build-up. To monitor and control energy deposition in a closed-loop treatment imaging system, magnetic resonance temperature-sensitive maps are obtained during and between each sonication for immediate feedback that allows real-time dosimetry.
In thermal ablative therapy, FUS-induced thermal energy is exploited to cause tissue coagulative necrosis. Above 57°C to 60°C over a few seconds, irreversible cell damage invariably occurs secondary to protein denaturing. The thermal ablation is nonselective and, therefore, both neoplastic and normal tissues are destroyed. Given the nonselective, heat-induced cell death, this treatment signals the need for a reliable and sensitive imaging method for monitoring tissue changes. As it can accurately detect temperature changes of less than 2°C to 3°C, magnetic resonance temperature-sensitive imaging (magnetic resonance thermometry) fulfills these criteria.
This all-or-none ablative effect of FUS irrespective of tissue type resembles surgical treatment. However, unlike surgery where the overlying tissue in the surgical path is destroyed, in FUS, the nonablated surrounding parenchyma is preserved. On the other hand, hyperthermia, similar to FUS, uses heat to cause its ablative effects on tissue. Unlike FUS, however, hyperthermia distributes relatively lower temperatures (43°C) over a longer period of time (30 to 60 minutes), thereby causing selective cell death of malignant cells as opposed to nontumoral cells that may survive the treatment. Other types of thermal ablative tool are the percutaneous heat-conducting probes, such as those used in radiofrequency ablation and laser therapy, that are obviously more invasive than FUS. Unlike FUS, which has a steep temperature gradient, heat-conducting probes cause a shallow temperature gradient when the heat is dissipated as it travels from the single-source probe. Probe-delivered thermal ablations, therefore, require long tissue exposures to increase the ablative volume and to reach sufficient thermal energy to cause cell death within the entire target volume. Additionally, unlike FUS, the probe ablation zone extends in each direction equally (spherically) and, therefore, cannot be tailored to fit the complex shape of a target volume. Underscoring its significance in thermal ablations, regardless of the ablative source, spatial monitoring and temporal monitoring of thermal heating are of utmost importance to avoid under- or overtreatment.
The nonthermal, nonablative effects of FUS are caused by multiple complex mechanisms, the most important being cavitation. Cavitation, defined as acoustically induced interactions from microscopic gas bubbles in the medium, results in the following: bubble oscillation, acoustic streaming, mechanical (acoustic radiation) forces, and inertial (transient) cavitation ( Fig. 2 ). Of these, inertial cavitation is believed to be responsible for most of the nonthermal biologic effects. In general, microbubbles are cavities filled with gas or vapor. More specifically, according to Minneart’s theory, a bubble is a spherical gas-filled structure in a liquid of constant hydrostatic pressure. Microbubbles can be seeded and induced by the ultrasound itself and generated within the native sonicated tissue or preformed microbubbles (clinically used ultrasound imaging contrast agents) that can be intravenously administered, the latter termed microbubble-enhanced therapeutic ultrasound. Preformed microbubbles are made from human serum albumin shells filled with perfluorocarbon gas perflutren (mean bubble diameter, 2.0 to 4.5 μm).
On interaction with an ultrasound beam and in response to a sound field, microbubbles, whether generated internally or administered iatrogenically, can cause any of the four previously mentioned biologic effects. Ultrasound bio-effects are either linear, stable, predictable, and controlled, or nonlinear, unstable, unpredictable, and uncontrolled, respectively. At low acoustic power, microbubbles oscillate and grow in size via rectified diffusion. Known as stable cavitation, this growth and oscillation contribute to nonthermal effects (the most important among them is disruption of the BBB) secondary to shear stresses on the cells caused by bubble oscillation, microstreaming, and radiation forces. Microstreaming, also termed acoustic streaming, refers to eddying circulation motions of fluid around the bubble. Radiation forces cause the bubble to move in the direction of the wave propagation, exerting mechanical force on and deformation of the endothelium perpendicular to the direction of the blood flow and length of the vessel. The shear stresses, resulting from bubble oscillation, microstreaming, and radiation forces, cause localized stretching of the cell membrane and stimulation of the endothelial cells via activation of stretch-sensitive ion channels, thereby inducing its biologic effects on the BBB. At high acoustic pressures, however, FUS causes oscillation and the rapid growth of bubbles, which, on implosion, unpredictably release stored energy in the form of shock waves. This violent collapse, also known as inertial cavitation, produces high-velocity jets and free radicals. The resulting biologic effects disrupt the cell membrane and endothelial tight junctions, allowing uncontrolled deposition of thermal heat. Nonlinear effects on heating can lead to hemorrhage and unwanted tissue destruction outside the focal area. The effects of cavitation, however, can be exploited in both thermal ablations and nonthermal treatments. In thermal ablations, cavitation-enhanced ablation causes the tissue to heat more rapidly to create a large volume of thermal ablation of a larger volume within relatively short treatment times. This latter method has been used in uterine fibroids. Both innate and preformed microbubbles allow BBB disruption (BBBD), to occur at lower energy levels. By acting as cavitation nucleation sites, preformed microbubbles further enhance the local ultrasound effects by dramatically lowering the ultrasound energy required to obtain the same amount of BBBD or tissue damage. Therefore, with the introduction of preformed contrast microbubble agents, the aforementioned adverse effects of uncontrolled heating, including hemorrhage and unwanted tissue destruction, will decrease.
Pretreatment Planning and Localization
Preprocedural planning is of utmost importance and involves the definition of the anatomy of the target lesion and adjacent structures, determination of the procedural approach, and calculation of a feasible trajectory for the delivery of sonications. MRI remains the imaging method of choice in all stages of an MRgFUS procedure, including preprocedural planning, intraprocedural monitoring, and postprocedural treatment validation, as it offers exquisite three-dimensional anatomic tissue characterization and functional mapping. Recently, at the Brigham and Women’s Hospital, the recommended MRI protocols have been released for all stages of an MRgFUS procedure. In the pretreatment stage, aside from the conventional MRI sequences, functional and advanced imaging techniques such as functional MRI (fMRI), perfusion imaging, and tractography are recommended. fMRI and tractography are important for functional assessment and preoperative planning, particularly in those patients in whom the tumor is adjacent or involving eloquent cortex and important white matter tracts, respectively. In addition to diffusion-weighted imaging, magnetic resonance perfusion imaging can be used to establish baseline and subsequently for magnetic resonance biomarkers to assess treatment response, especially in those patients whose radiological picture can be confounded on the administration of adjuvant chemotherapy and radiation.
Intraprocedure Targeting and Monitoring
Once the transcranial apparatus, consisting of the hemispherical phased-array transducer and underlying cranial cooling system, is secured and the patient is in positioned in the MRI suite, an immediate pretreatment MRI is obtained for retargeting the preselected lesion and re-evaluating the perilesional parenchyma. Both magnetic resonance thermometry and conventional and advanced MRI techniques are pivotal.
MRI thermometry remains the imaging modality of choice for intraprocedural monitoring and establishing treatment conclusion. Using proton resonance frequency shifts, magnetic resonance thermometry detects with great precision minute changes in temperature caused by focused sonications, and it can be used as a surrogate for tissue viability. At the onset of the procedure, magnetic resonance thermometry confirms the correct focal point on the target lesion by detecting noncoagulative tissue changes caused by low-power sonications. Subsequently, magnetic resonance temperature-sensitive measurements are obtained during and between each high-power therapeutic sonication. Therefore, MRI thermometry, unlike other imaging modalities, allows for a controlled energy deposition by providing immediate real-time dosimetry and permitting real-time intra-procedural closed-loop feedback of thermal ablative procedures.
Conventional and, depending on the situation and tumor type, advanced MRI sequences obtained intraoperatively provide real-time updates on the progress and extent of tumor ablation. MRI sequences have been put forth by the Brigham and Women’s Hospital, and they are tailored to confer the least amount of intraprocedural acquisition time. As such, the acquisition of postcontrast T1 weighted imaging in enhancing high-grade tumors and fluid-attenuated inversion recovery (FLAIR) sequences in both high- and low-grade tumors/gliomas is used to determine the extent of ablation. This evaluation is important, as the subtotal removal remains one of the single most important independent predictors of tumor recurrence and, therefore, poor prognosis.
Postprocedure Validation
Once the desired therapeutic endpoint is achieved, postcontrast MRI is acquired for treatment validation and to establish a post-treatment baseline MRI. In thermal ablations, a focal region of nonenhancement reflects a positive response to ultrasound-induced thermal ablation and corresponds to tissue necrosis and blocked capillaries. In nonthermal therapy such as with disruption of the BBB, enhancement of the sonicated area reflects increased permeability, confirming a positive response to therapy. MRI also can be used to document postprocedure perilesional edema that progressively resolves after 48 to 74 hours.
Principles of MRgFUS
MRgFUS combines and integrates a therapeutic and imaging modality, the FUS device, and MRI, respectively, into a single image-guided therapy delivery system. FUS, the therapeutic instrument, causes direct ultrasound-induced nonthermal (nonablative) and thermal (ablative) changes in the target tissue. As an ablative modality, FUS’ effects on tissue are caused by thermal heating. As a nonablative tool, FUS can reversibly disrupt the BBB, resulting in an increase in cerebrovascular capillary permeability and a consequent increase in the flux of relatively larger, nonlipophilic, and high molecular weight molecules that otherwise would not cross the BBB. Other nonthermal effects include an increase in cell membrane permeability for use in gene therapy and neuromodulation.
Ultrasound penetrates through tissues without depositing any significant amount of energy. The focusing of ultrasound beams results in a concentration of energy at the focal point. At a given absorption coefficient corresponding to the specific tissue, in thermal ablations, the generation of thermal energy is maximal at the point of convergence where the summation of the acoustic intensity is highest. Overlapping sonications are delivered to the target tissues, generating heat at the focal point that exceeds a 60°C thermal coagulation threshold. FUS induces thermal coagulative necrosis of approximately 1 to 3 mm in diameter (perpendicular to the beam) and about twice as long in length (parallel to the beam). Given the small focal point per sonication, multiple strategies are employed in an effort to increase the focal point size and decrease overall treatment time. Strategies to increase the ablation volume include “cavitation-enhanced ablation” and treating multiple sites simultaneously.
Before delivering the sufficient treatment doses, subthreshold, noncoagulative low-power sonications are delivered to identify the location of the focus and to provide correct targeting of the preselected location or lesion. Magnetic resonance thermometry confirms the focal point on the target lesion by detecting only short and small temperature elevations below 60°C at a time when there are no tissue changes. After this targeting confirmation, high-power focused sonications (500 to 20,000 W/cm 2 ) of short duration (1 to 60 seconds) are administered. Because of the relatively long cooling time (over minutes) between sonications, the overall treatment time is lengthy. Cooling is necessary to avoid the summation of heat effects, which, without cooling, can result in larger tissue volumes than the focal volume. Unlike probe-delivered thermal ablations with wide gradients, FUS has steep thermal gradients. Short sonication times prevent substantial cooling effects from blood flow and perfusion and simultaneously reduce heat build-up. To monitor and control energy deposition in a closed-loop treatment imaging system, magnetic resonance temperature-sensitive maps are obtained during and between each sonication for immediate feedback that allows real-time dosimetry.
In thermal ablative therapy, FUS-induced thermal energy is exploited to cause tissue coagulative necrosis. Above 57°C to 60°C over a few seconds, irreversible cell damage invariably occurs secondary to protein denaturing. The thermal ablation is nonselective and, therefore, both neoplastic and normal tissues are destroyed. Given the nonselective, heat-induced cell death, this treatment signals the need for a reliable and sensitive imaging method for monitoring tissue changes. As it can accurately detect temperature changes of less than 2°C to 3°C, magnetic resonance temperature-sensitive imaging (magnetic resonance thermometry) fulfills these criteria.
This all-or-none ablative effect of FUS irrespective of tissue type resembles surgical treatment. However, unlike surgery where the overlying tissue in the surgical path is destroyed, in FUS, the nonablated surrounding parenchyma is preserved. On the other hand, hyperthermia, similar to FUS, uses heat to cause its ablative effects on tissue. Unlike FUS, however, hyperthermia distributes relatively lower temperatures (43°C) over a longer period of time (30 to 60 minutes), thereby causing selective cell death of malignant cells as opposed to nontumoral cells that may survive the treatment. Other types of thermal ablative tool are the percutaneous heat-conducting probes, such as those used in radiofrequency ablation and laser therapy, that are obviously more invasive than FUS. Unlike FUS, which has a steep temperature gradient, heat-conducting probes cause a shallow temperature gradient when the heat is dissipated as it travels from the single-source probe. Probe-delivered thermal ablations, therefore, require long tissue exposures to increase the ablative volume and to reach sufficient thermal energy to cause cell death within the entire target volume. Additionally, unlike FUS, the probe ablation zone extends in each direction equally (spherically) and, therefore, cannot be tailored to fit the complex shape of a target volume. Underscoring its significance in thermal ablations, regardless of the ablative source, spatial monitoring and temporal monitoring of thermal heating are of utmost importance to avoid under- or overtreatment.
The nonthermal, nonablative effects of FUS are caused by multiple complex mechanisms, the most important being cavitation. Cavitation, defined as acoustically induced interactions from microscopic gas bubbles in the medium, results in the following: bubble oscillation, acoustic streaming, mechanical (acoustic radiation) forces, and inertial (transient) cavitation ( Fig. 2 ). Of these, inertial cavitation is believed to be responsible for most of the nonthermal biologic effects. In general, microbubbles are cavities filled with gas or vapor. More specifically, according to Minneart’s theory, a bubble is a spherical gas-filled structure in a liquid of constant hydrostatic pressure. Microbubbles can be seeded and induced by the ultrasound itself and generated within the native sonicated tissue or preformed microbubbles (clinically used ultrasound imaging contrast agents) that can be intravenously administered, the latter termed microbubble-enhanced therapeutic ultrasound. Preformed microbubbles are made from human serum albumin shells filled with perfluorocarbon gas perflutren (mean bubble diameter, 2.0 to 4.5 μm).
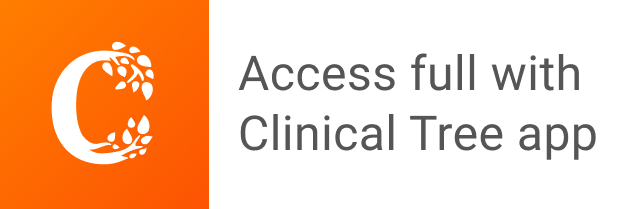