11
Gastrointestinal Brachytherapy
Alexandra J. Stewart, Nikolaos Tselis, Michele Albert, Nitika Thawani, and Arthur Sun Myint
Brachytherapy in gastrointestinal cancer is generally underutilized but its uptake is increasing as more phase III evidence is becoming available for its use and mechanisms of administration are becoming easier and more reproducible. This chapter covers brachytherapy from esophagus to anus including 50 kV contact brachytherapy and injectable microspheres.
ESOPHAGEAL CANCER
Esophageal cancer is the eighth most common cancer worldwide, comprising 4% of the total cancer incidence (1). Eighty-three percent of these cases were diagnosed in the developing world. Predisposing factors include tobacco and alcohol use (predominantly squamous cell carcinoma) and reflux and obesity (predominantly adenocarcinoma). Esophageal cancer is the sixth most common cause of cancer death, with mortality following geographical patterns for incidence.
There will be an estimated 17,000 patients with esophageal cancer in the United States in 2015. In the United States, the incidence of adenocarcinoma of the esophagus is increasing, whereas that of squamous cell carcinoma is decreasing slightly. Despite the accessibility of the organ, only 6% of esophageal cancers receive brachytherapy, according to the Patterns of Care Study (2).
Esophageal brachytherapy can be administered in the radical and palliative settings. Radically, it can be a single modality treatment for very early tumors or as definitive management of nonsurgical candidates. The patient is consented for an endoscopic assessment. The patient may choose local pharyngeal anesthesia or sedation for the procedure. The endoscopist examines the tumor using a gastroscope and documents the distance of the tumor from the teeth and the length of the visible tumor. Radiopaque clips or markers are placed at the superior and inferior intraluminal extent of the tumor. The brachytherapy catheter with a diameter of 0.6 to 1.0 cm (Figure 11.1) can be sited via the nasal or oral route. A thinner catheter would lead to a higher mucosal dose, and therefore, higher toxicity. Use of thicker catheters has shown a low incidence of toxicity (3). When sited orally, a guidewire is passed down the side port of the endoscope past the tumor and the brachytherapy catheter is passed over the guidewire traversing the tumor. If sited nasally a guidewire can be passed first to aid passage via the nasopharynx. The catheter is passed at least 4 cm distal to the tumor and firmly secured at the cheek or nose. It may help to mark the brachytherapy catheter with 1 cm interval markings to aid catheter placement if it is not premarked by the manufacturer.
Figure 11.1 Single-channel esophageal HDR brachytherapy applicator (Elekta, Stockholm, Sweden). HDR, high dose rate.
In the setting of a high-grade obstruction, a more complex approach is indicated. The goal is to have the esophageal applicator placed through the nose. If the patient has a gastrostomy tube, the procedure can be done quite easily. The applicator can be passed through the nose and led out of the mouth. Then the endoscope is passed through the mouth and advanced, until the gastrostomy tube is visualized. A long suture or snare should be inserted into the gastrostomy tube and grasped by the endoscope. The endoscope should be withdrawn, pulling the suture out through the patient’s mouth, while care must be taken to have the other end of the suture secure outside the gastrostomy tube. The suture (or snare) should be attached to the weighted end of the esophageal catheter and slowly pulled from the gastrostomy tube while advancing the catheter into the patient’s mouth. If the endoscope cannot pass the obstruction, an additional step of passing a thin biopsy catheter past the lesion may work, thereby grabbing the snare, which, in turn, will be used to draw the applicator safely down. Care must be taken so as not to disrupt the mucosa or to create a false lumen. These procedures require a high level of endoscopic skill and carry significant potential risk of bleeding, mediastinitis, and infection, and belong in tertiary referral centers with significant case loads.
Once the patient has recovered from the endoscopy, he or she is transferred for imaging to determine the catheter position. Although this can be performed using conventional X-ray simulation (Figure 11.2) it is preferable to use CT scanning (Figure 11.3). The scan will determine the catheter position and also show whether there is extraluminal extension of the disease outside the area marked by the clips. With this taken into account, the treatment volume should extend 2 cm superior and inferior to clips and all macroscopic disease to encompass any microscopic tumor foci. Treatment is generally prescribed at 1 cm from the source axis, often using straight-line calculations to allow rapid treatment to prevent patient discomfort. Three-dimensional (3D) planning can be used for complex cases and the dose received by organs at risk determined; this may also be preferable in radical cases. It is important to note that the scanning position should be replicated for treatment to more accurately replicate the predicted dose to organs at risk (OAR).
Figure 11.2 Anteroposterior conventional simulator radiograph demonstrating brachytherapy catheter in situ with clinical target volume and offset marked in red.
Figure 11.3 Sagittal CT image of the thorax demonstrating the isodose curves for an esophageal brachytherapy plan prescribed at 1 cm. This was a customized plan taking catheter curvature into account.
The best role for the addition of brachytherapy for esophageal cancer would be in Stage I tumors. Superficial tumors, described as being only mucosal or submucosal, have shown responses and low toxicity (9−11). However, the use of brachytherapy as a sole radical treatment modality is controversial as The Japanese Society for Therapeutic Radiation Oncology released a contradictory study that indicated that, for superficial lesions, brachytherapy had no benefit over external beam radiotherapy (EBRT) (12). However, most studies show a benefit with low toxicity, especially for the mucosal lesions.
Brachytherapy can be used to deliver a highly localized esophageal boost following radical EBRT. The tumor response in radical schedules has been shown in a small radiobiological analysis to be related to dose with a brachytherapy biological effective dose (BED) higher than 28 Gy in addition to 44.2 Gy EBRT yielding a significant increase in survival (13). The α/β ratio used for BED calculation was not given but the reported BEDs would be consistent with a higher α/β ratio representing acute toxicity or tumor control. Brunner et al examined chemoradiotherapy with a concomitant boost delivering up to 64.8 Gy EBRT plus up to 18 Gy in three fractions of brachytherapy (14). Survival was 61% at 1 year and 18% at 3 years. The fistula rate was 12% but it was tumor related in 8%. Aggarwal et al used hypofractionated EBRT in combination with 10 to 15 Gy single-fraction esophageal brachytherapy in patients unfit for surgical excision or chemoradiotherapy and demonstrated 51% 1-year survival (15). There were no fistulae and 8% of patients developed benign strictures. Calais et al added Mitomycin-C with cisplatin and 5-fluorouracil (5-FU) to 60 Gy EBRT and 10 Gy in two fractions of brachytherapy (16). The 3-year local control was 57% with 11% long-term toxicity.
Two randomized trials have examined the role of a brachytherapy boost in radical treatment. Yin studied 200 patients (17). The dose delivered by external beam radiation alone was 70 Gy in 35 fractions compared with 50 Gy external beam in 25 fractions plus three to four applications of medium dose rate (MDR) brachytherapy for a dose of 19.6 to 26.16 Gy. The 5-year local control was improved in the brachytherapy arm, 61.3% versus 43%, and 5-year overall survival was also improved, 17% versus 10% (P < .05). The toxicity was reported to be the same, 12.6% of perforation or hemorrhage in either arm. A second randomized study by Sur et al had similar results for 50 patients (18). The dose prescribed was 55 Gy EBRT versus 35 Gy EBRT and 12 Gy HDR boost in two fractions 1 week apart. The complication rate was higher for strictures in the brachytherapy arm, 8% versus 4%.
However, dose escalation needs to be undertaken with care following the results of the Radiation Therapy Oncology Group (RTOG) 92–07 trial. This was a phase I/II trial investigating the feasibility and tolerance of EBRT, brachytherapy, and concurrent chemotherapy using cisplatin and 5-FU. Initial use of LDR was discontinued due to poor uptake and the HDR dose decreased from 15 Gy in three fractions to 10 Gy in two fractions following unacceptable rates of toxicity; after 70% of the patients were added at the higher HDR dose, a large perforation rate was noted: 58% grade 3, 26% grade 4, and 8% grade 5 (fatal). None of the lower dose HDR patients had grade 4 or 5 toxicity. Complete remission rates were 74% following EBRT and brachytherapy with a 1-year survival of 49% (19). However, the local control was 37%. On the basis of the low local control and high toxicity, the study did not progress to phase III. This study used more chemotherapy, smaller catheters, and higher doses of brachytherapy than other studies. This could explain the weaker mucosa and higher perforation rates. Table 11.1 shows the toxicity and fraction schema of several studies. Caution was urged when using radical treatment schedules employing brachytherapy and chemotherapy. Thus, the American Brachytherapy Society (ABS) recommended 10 Gy prescribed at 1 cm in two fractions 1 week apart following chemoradiotherapy 45 to 50 Gy or radiotherapy alone up to 60 Gy (20).
Table 11.1 Table of toxicity for esophageal endoluminal brachytherapy
For palliation, esophageal brachytherapy delivers improvement of swallowing in the majority of cases, with maintenance of swallowing in most of the remainder. Two randomized trials have examined the role of brachytherapy versus esophageal stenting. Homs et al randomized 209 patients and demonstrated improved efficacy of brachytherapy 12 Gy in one fraction over stenting (21) with improved quality of life, longer duration of symptom palliation, and equivalent overall health care costs (22). As there is a slight delay in onset of symptom palliation with brachytherapy, it was recommended that patients with a predicted survival of less than 2 months have stenting alone. In patients with predicted survival of more than 2 months, brachytherapy should be used instead of stenting. Berquist et al randomized 65 patients to fractionated brachytherapy using 21 Gy in three fractions versus stenting. No difference in dysphagia relief was seen at 6 months, though an overall improvement in speech was seen in the brachytherapy group at that time point. The number of patients in the trial may have been too small to draw definitive conclusions—there were only 14 patients alive at the 6 month follow-up point (23). A small randomized study of 41 patients randomized patients to brachytherapy with or without stent insertion. Stenting plus brachytherapy provided more immediate relief of dysphagia but at 7 weeks there was no difference in dysphagia between the two groups (24).
A Cochrane meta-analysis in 2014 showed that brachytherapy has a longer duration of palliation than stenting with significantly better quality of life and lower complications (33% vs 47% [25]) and may even provide a survival advantage. Brachytherapy plus EBRT shows a trend toward better quality of life and improved dysphagia. Brachytherapy was seen to be comparable to laser for palliation and may improve the dysphagia-free interval when EBRT is added to the combination of laser and brachytherapy. The quality of life also appears to be improved in this setting (26).
An International Atomic Energy Agency (IAEA) randomized study showed that for patients with obstructive squamous cell carcinoma, the addition of EBRT 30 Gy in 10 fractions to 16 Gy in two fractions of brachytherapy resulted in significantly better symptom relief than brachytherapy alone with dysphagia relief of 83% versus 67% at 100 days (27). This benefit was maintained at the 300 day follow-up point. There was no difference in overall survival between the two groups. Whether the EBRT course could further be shortened is currently under investigation.
The ABS selection criteria for esophageal brachytherapy are listed in Table 11.2 (20). A range of doses for HDR esophageal brachytherapy is considered acceptable. The ABS recommended dose schema can be seen in Table 11.3. A dose comparison study of 18 Gy in three fractions versus 16 Gy in two fractions by the IAEA (28) showed no difference in efficacy or toxicity with an overall 11% stricture rate and 10% fistula rate—all due to disease progression. The randomized trial showing a significant benefit for brachytherapy used 12 Gy in one fraction prescribed at 1 cm (21).
Table 11.2 American Brachytherapy Society selection criteria for brachytherapy in the treatment of esophageal cancer
Good candidates Primary tumor ≤ 10 cm in length Tumor confined to esophageal wall Thoracic esophagus location No regional lymph node or systemic metastasis |
Poor candidates Extraesophageal extension Tumor > 10 cm in length Regional lymphadenopathy Tumor involving gastroesophageal junction or cardia |
Contraindications Esophageal fistula Cervical esophageal location Stenosis that cannot be bypassed |
Source: Adapted from Ref. (20). Gaspar LE, Nag S, Herskovic A, et al. American Brachytherapy Society consensus guidelines for brachytherapy of esophageal cancer. Clinical Research Committee, American Brachytherapy Society, Philadelphia, PA. Int J Radiat Oncol Biol Phys. 1997;38(1):127–132.
Table 11.3 American Brachytherapy Society–suggested schema for esophageal brachytherapy
All doses specified at 1.0 cm from mid-source or mid-dwell position |
Radical |
External beam radiation 45–50 Gy in 1.8–2.0 Gy/fraction, 5 fractions/wk, wk 1–5 Brachytherapy High dose rate—total dose of 10 Gy, 5 Gy/fraction, 1 fraction/wk, starting 2–3 wk following completion of external beam Low dose rate—total dose of 20 Gy, single course, 0.4–1.0 Gy/hr, starting 2–3 wk following completion of external beam |
Palliative |
Recurrent after external beam radiation or short life expectancy Brachytherapy High dose rate—total dose of 10–14 Gy, 1 or 2 fractions Low dose rate—total dose of 20–40 Gy, 1 or 2 fractions, 0.4–1.0 Gy/hr |
No previous external beam radiation External beam radiation 30–40 Gy in 2 to 3 Gy fractions Brachytherapy High dose rate—total dose of 10–14 Gy, 1 or 2 fractions Low dose rate—total dose of 20–25 Gy, single course, 0.4–1.0 Gy/hr |
No previous external beam radiation, life expectancy > 6 mo External beam radiation 45–50 Gy in 1.8–2.0 Gy/fraction, 5 fractions/wk, wk 1–5 Brachytherapy High dose rate—total dose of 10 Gy, 5 Gy/fraction, 1 fraction/wk, starting 2–3 wk following completion of external beam Low dose rate—total dose of 20 Gy, single course, 0.4–1.0 Gy/hr, starting 2–3 wk following completion of external beam |
PANCREATIC CANCER
Pancreatic cancer comprises 2% of the worldwide cancer incidence and yet accounts for 4% of worldwide mortality from cancer (1). Patients often present with inoperable disease at an advanced age and curative treatment is rare with high associated morbidity. Brachytherapy is rarely used in pancreatic cancer with the mainstays of treatment being surgery for cure or chemotherapy for palliation. One reason for this is the fear of pancreatic fistula or pancreatitis during or after brachytherapy treatment.
A series of 12 patients receiving EBRT and 125I seed implants, placed operatively under direct vision, showed favorable local control with only one patient recurring locally and good symptom palliation (32). There were two cases of gastric hemorrhage and two pancreatic fistulae, which resolved spontaneously. An enhanced series from the same institution describes 98 patients who received operative 125I implants, 27 of whom received EBRT, and 27 also received chemotherapy (33). Postoperative complications occurred in 19 patients, predominantly related to the surgical procedure. Median survival was 7 months overall but in stage T1 patients an improved survival of 18.5 months was seen. Palladium-103 (103P) intraoperative implants were also used with similar outcomes and possibly lower toxicities (34,35). However, this form of brachytherapy was an invasive, technically specialized technique that has not had widespread uptake. It is likely with the continued development of stereotactic body radiation therapy that the need for pancreatic brachytherapy will further diminish.
The use of wires loaded with radium-224 (224Ra) has been investigated in vitro and in vivo in murine pancreatic cancer models and shows promising results for local control (36). The use of stents loaded with 125I seeds placed into pigs’ normal pancreatic ducts was investigated. No complications were seen as a result of stent placement, meriting further investigation of this as a potential treatment modality (37).
BILIARY TRACT CARCINOMA
Cholangiocarcinoma is rare but still accounts for 1% of worldwide cancer deaths (1). Treatment is often limited by the location of the tumor or advanced patient age, with an average age of diagnosis being 65 years. Surgical excision carries a high mortality and a 5-year survival rate of only 11% to 44% depending on the site of lesion within the biliary tree (38). Therefore, strategies that provide radical treatment where surgery is not anatomically possible or palliation for patients who are not fit for surgery are desirable.
The brachytherapy catheter is optimally placed percutaneously under radiographic guidance. It is best to place the catheter within a stent, which allows localization of the lesion (Figure 11.4A) and helps to prevent biliary stenosis as a consequence of radiotherapy, although late strictures still remain a high risk. When administered radically, brachytherapy tends to be used in combination with EBRT. The patient undergoes a CT planning scan with the brachytherapy catheter in situ. A brachytherapy boost volume (Figure 11.4B) and a wider EBRT field (Figure 11.4C) are determined using the brachytherapy catheter as a guide. Dose and fractionation of each modality are then determined using the tolerance of the organs at risk, namely whole liver, right kidney, and duodenum. The brachytherapy boost is optimally administered first to decrease the risk of sepsis and the catheter removed for the subsequent EBRT. Suggested contraindications to treatment are a bilirubin level greater than 2.5 times the upper limit of normal following drainage, more than five liver metastases, uncorrectable impaired coagulation, or massive ascites (39).
Figure 11.4 (A) X-ray image showing radiopaque biliary stents with a brachytherapy catheter in situ. A marker wire is placed inside the brachytherapy catheter to define its position. (B and C) The same axial CT image of the upper abdomen showing the brachytherapy catheter pictured in (A) within the liver. The clinical target volume is delineated in bold red. The brachytherapy isodoses (B) and IMRT isodoses (C) are pictured with the 100% isodose line in narrow red. Organs at risk have been delineated. IMRT, intensity modulated radiation therapy.
When administered as a radical treatment, sole therapy with brachytherapy generally resulted in locoregional failure in surrounding bile ducts (though these series were in the pre-CT planning era); therefore, brachytherapy was combined with EBRT and gave survival that was improved over that expected in nonirradiated patients. Older series used LDR in the form of radium-228 (228Ra) (40) or iridium-192 (192Ir) (41,42). More recent series utilize HDR (43−46) usually in combination with EBRT and metal biliary stents. One retrospective series suggested that the addition of a brachytherapy to EBRT gave a survival advantage (0% vs 20% at 2 years [45]). Dose appears to be important with patients receiving a combined dose above 55 Gy having better outcomes (47). Complications of treatment include cholangitis and obstructive jaundice, hence the uptake of concurrent biliary stenting. A 5-year survival rate of 10% in one series (44) is favorable in comparison to surgical outcomes (38) especially when it is considered that these patients were unfit for surgery. In addition, a number of these series had small cohorts of long-term survivors following radiotherapy (5 years plus) who were alive with no evidence of disease. Lower doses of brachytherapy alone have been used for less fit patients in the palliative setting (48) and appear to help maintain stent patency over stenting alone (49).
The role of adjuvant brachytherapy following surgical excision is unclear with several large series showing a significant survival advantage using radiotherapy (usually using a combination of EBRT and brachytherapy) particularly in the setting of R1 resection (48). However, in a series of 196 patients with perihilar tumors who were surgically operable, adjuvant brachytherapy alone was administered to 45% of patients but was not seen to affect survival on multivariate analysis (38).
LIVER TUMORS
According to World Health Organization (WHO) estimations, liver cancer is the fifth most common cancer type among men (7.5% of all male malignancies) and the seventh among women (3.4% of all female malignancies), whereas it accounted worldwide for approximately 746,000 deaths in 2012 (primary and secondary liver cancer), being the second cause of all tumor-associated deaths (50). Primary hepatocellular carcinoma (HCC) is increasing in incidence, and it is now the sixth most common cancer worldwide (1), with particularly high prevalence in Southeast Asia. It is associated with hepatitis B and C virus infection (85% of international incidence [51]), alcohol intake (52), and foods contaminated with aflatoxin (53). Because of its high fatality rates, the incidence and mortality rates are almost equal (54). HCC is the third most common cause of death from cancer worldwide; hence, improved treatment strategies are widely sought after. Complete surgical resection remains the gold standard for the cure of primary hepatic cancer (55); however, a large subgroup of patients is not suitable for an open surgical approach and the recurrence rate after partial hepatectomy is more than 50% (56). Therefore, strategies to improve surgical cure rates are under investigation (57).
In patients with unresectable disease, hyperthermal techniques such as radiofrequency ablation (RFA) and laser-induced thermotherapy have been reported as alternative local treatment choices (58−60). However, one of the major challenges with these approaches is incomplete treatment of large tumors due to their proximity to large volume vascular structures resulting in residual cancer tissue. Factors impairing the therapeutic ratio are tumor size, with an accepted upper size limit of 3 to 4 cm for optimal treatment, and the “heat sink” effect of nearby vasculature, stopping effective cytoreduction in perivascular lesions (61). To overcome these limitations, hyperthermal ablative therapies have been combined with other treatment modalities such as transarterial chemoembolization (TACE [62]), percutaneous ethanol injection (PEI [63]), or radiotherapy. Lin et al combined RFA with MRI-guided percutaneous implantation of 125I seeds to give a complete response rate of 96% in lesions that would otherwise not be fully covered by RFA (64). In addition to use in the adjuvant setting (65), LDR brachytherapy has also been employed as the sole treatment for unresectable primary liver malignancies. Lin et al treated 65 inoperable hepatocellular carcinoma lesions using MRI-guided percutaneous implantation of 125I seeds (66). The minimum peripheral dose delivered was 144 Gy and the treatment yielded an overall response rate of 84.5% with no clinically relevant complications.
CT-guided interstitial HDR brachytherapy has been used in a similar setting for the safe treatment of extensive hepatocellular carcinoma lesions unsuitable for surgery or thermal ablation. Catheter placement is performed employing CT guidance under local or general anesthesia. The maximum insertion depth, direction, and position of the catheters are estimated by interactive CT scanning with their number, geometrical alignment, and distance depending on the size and shape of the tumors. Round-tipped plastic catheters, usually 6 French (2 mm) outer diameter and 200 mm long, are implanted percutaneously through skin incisions under breath hold (Figure 11.5A). A rigid tungsten alloy central obturator is employed to maintain catheter integrity and stability during percutaneous transhepatic insertion.
After completion of catheter implantation, a contrast-enhanced spiral CT of the liver (slice thickness of at least 3 mm) is acquired for CT-based 3D treatment planning with anatomy-oriented dose optimization (Figure 11.5B). Tumor demarcation with corresponding target volume delineation is performed using image fusion with a contrast-enhanced liver MRI scan. The dose distribution is normalized to the calculated mean dose value on the tumor surface and the reference dose specified at the 100% isodose surface (Figure 11.5C). The dose constraints ensure that no more than 33% of the liver parenchyma receives more than 5 Gy. The entire implantation–planning–optimization procedure requires approximately 45 to 60 minutes, following which brachytherapy can be delivered. Treatments are administered over consecutive days with an interfraction interval of at least 6 hours or as single-fraction schedules using an 192Ir HDR afterloading system.
Figure 11.5 (A, B, and C) Forty-seven-year-old woman with hepatocellular carcinoma of the left lobe. The patient received CT-guided interstitial HDR brachytherapy after failure of systemic tyrosine kinase inhibitor treatment while refusing hepatectomy. The brachytherapy was delivered in a single fraction of 15 Gy. (A) Axial image-fusion of the postimplant CT dataset with a preinterventional contrast-enhanced liver MRI for optimal tumor demarcation. The single interstitial catheter is identifiable in the central tumor portion by radiopaque markers along its axis. (B) Three-dimensional implant reconstruction for CT-based treatment planning showing the liver tumor with the implanted catheter. The volumetrically calculated lesion size was 103 cm3. (C) Axial CT/MRI-image fusion illustrating the dose distribution of the implant for a plane lying centrally to the target extension. The tumor is delineated in red (PTV). Minimal dose per fraction is the reference dose of 15 Gy, specified as the 100% isodose. The isodose color code convention is: dark red = 300% (isodose = 45 Gy); pink = 200% (isodose = 30 Gy); yellow = 150% (isodose = 22.5 Gy); orange = 100% (isodose = 15 Gy); and green = 50% (isodose = 7.5 Gy). The central tumor portion is covered at least by the 300% isodose and receives doses higher than 45 Gy within a single HDR treatment fraction. HDR, high dose rate; PTV, planning target volume.
Consistent and reproducible 9 to 12 month local control rates of 80% to 96% have been reported for patients with inoperable primary liver tumors (Table 11.4). Collettini et al treated 212 lesions ranging from 1.8 to 12 cm (mean: 5 cm) in 98 patients with hepatocellular carcinoma (67). Treatment consisted of CT-guided HDR brachytherapy with a mean minimum tumor-enclosing dose of 16.51 Gy. During the follow-up period (20 months), a total of 18 of 212 (8.5%) tumors recurred, yielding a local control of 21.1 months, with one self-resolving subcapsular hematoma recorded. Mohnike et al reported results of 140 lesions in 83 patients with hepatocellular carcinoma treated with HDR brachytherapy of 15 to 25 Gy using the same CT-guided interstitial technique (68). The local control rate was 95% at 12 months with 7.2% complications requiring intervention.
Table 11.4 Literature results of CT-guided interstitial for primary and metastatic liver malignancies
The majority of cancer affecting the liver is that of metastatic disease. Potentially curative treatment can be offered to selected patients who have liver-only disease, a limited number of surgically resectable metastases and a favorable response to chemotherapy (74). Patients who are not cured still experience longer overall survival compared to those with unresected disease (75,76). Therefore, treatment of individual metastases may offer improved survival and should be considered. As in the treatment of primary hepatic malignancies, CT-guided HDR brachytherapy has been described for the interstitial irradiation of extensive metastatic tumors. In contrast to hyperthermal modalities, it allows precise predictable energy deposition regardless of tissue inhomogeneity, thermal conductivity, or tumor perfusion, generating 9 to 12 month local control rates of 79% to 93.5% (Table 11.4). Collettini et al reported results from 80 patients with 179 colorectal liver metastases with a largest diameter of 11 cm, treated to 20 Gy in a single fraction. At a median follow-up of 17 months, local control was 87% (77). Wieners et al reported on 115 unresectable breast cancer metastases with a median tumor size of 4.6 cm (1.5–11 cm) in 41 patients (72). Treatment consisted of a single fraction of HDR brachytherapy with a median prescribed dose of 18.5 Gy (range: 12–25 Gy), which yielded local control and overall survival rates of 93.5% and 79%, respectively, at 12 months. The authors documented 1.4% toxicity necessitating intervention and 8.6% complications that were self-limiting.
The use of permanent interstitial LDR brachytherapy has been described for unresectable microscopic or gross residual metastatic liver disease. Nag et al treated 64 patients with incomplete resection of hepatic metastases by intraoperative 125I seed implantation with a median minimum peripheral dose of 160 Gy (78). The median implant volume was 16 cm3 with overall local control rates of 44% and 22% at 12 and 36 months, respectively, and a 5-year survival of 38% in those with solitary metastases.
Stereotactic body radiation therapy has emerged as a noninvasive technique for the ablation of unresectable liver malignancies. Treatment schedules include either hypofractionated or single-fraction regimens generating local control rates from 55% to 100% at 24 months for lesions predominantly less than 4 cm (79−81). In HDR brachytherapy series, the reported median tumor diameters are in the range of 3.1 to 7.1 cm with median lesion volumes of 61 to 99 cm3 (Table 11.4). As such, interstitial HDR brachytherapy can be an effective alternative for patients who are not candidates for stereotactic body radiation therapy due to large tumor size or when stereotactic radiation delivery systems are not available.
Selective internal radiation therapy (SIRT) can also be used for the treatment of primary and secondary liver tumors and may improve survival time and decrease symptoms (82). It may also shrink larger primary liver tumors to enable hepatic transplantation (83). In contrast to normal liver, which receives the majority of its blood supply from the hepatic portal system, tumors within the liver receive the majority of their blood supply from the hepatic artery. SIRT takes advantage of this distribution to deliver β radiation in a highly localized distribution within the tumor. Yttrium-90 (90Y) is bound to resin (SirSpheres, Sirtex Medical Inc, Sydney, Australia) or glass (TheraSpheres, BTG International Canada Inc, Ottawa, Canada) microspheres and injected via a catheter directly into the arterial supply of the liver (Figures 11.6A and B). The treatment is administered using a closed circuit system, which is specific to each manufacturer. The majority of the dose is delivered within 11 days of the procedure. When used for metastatic disease, the patient should have multiple unresectable liver-predominant disease and the procedure should be carefully timed in conjunction with systemic chemotherapy. The bilirubin should be less than two times the upper limit of normal. By diagnostic mesenteric angiography, the liver and tumor vasculature can be mapped and coils placed in nontarget sites to embolize them. Doses can be calculated using one of two methods, the empiric method (Table 11.5) is based on the volume of liver that the tumor occupies. The body surface area (BSA) method calculates the dose using the following equation:
Figure 11.6 (A) A 55-year-old woman with a diagnosis of multifocal hepatocellular carcinoma (histologically proven) with no overt cirrhosis. MRI images with gadoxetic acid contrast demonstrating the appearances of the tumor at baseline (i, ii) and follow-up at 3 months (iii, iv) and 18 months (v, vi) after 90Y radioembolization. Figures i, iii, and v display the arterial phase, and ii, iv, and vi the hepatobiliary phase. The image series displays at baseline a multifocal bilobar disease with a partial response after 3 months and a complete remission of the disease (according to perfusion criteria) after 18 months. The hyperintense elliptical mass at the right lateral liver capsule resembles a persisting hematoma, which was related to a liver biopsy. (B) A 69-year-old woman with synchronous hepatic metastatic colorectal cancer, first diagnosed 2 years prior to presentation for a 90Y radioembolization. In that period, the patient received all standard palliative chemotherapy including antibodies. However, the disease progressed on standard chemotherapy and further therapy was warranted. MRIs with gadoxeticacid contrast are demonstrated at baseline (i–iii), at 3 months (iv–vi), and at 12 months (vii–ix) after 90Y radioembolization. Series i–iii displays hepatobiliary phase imaging, series iv–ix display portal venous phase imaging. The image series display at baseline a multifocal bilobar disease with a beginning partial response after 3 months and a further remission of the disease after 12 months. No concomitant chemotherapy was administered during this follow-up period. A relapse of the hepatic disease occurred 18 months after radioembolization. Salvage chemotherapy was recommenced and the patient died 34 months after radioembolization.
Table 11.5 The empiric method of dose calculation for selective internal radiation therapy
Estimated Degree of Tumor Involvement in the Liver (%) | Recommended 90Y Amount for Treatment (GBq) |
< 50 | 3.0 |
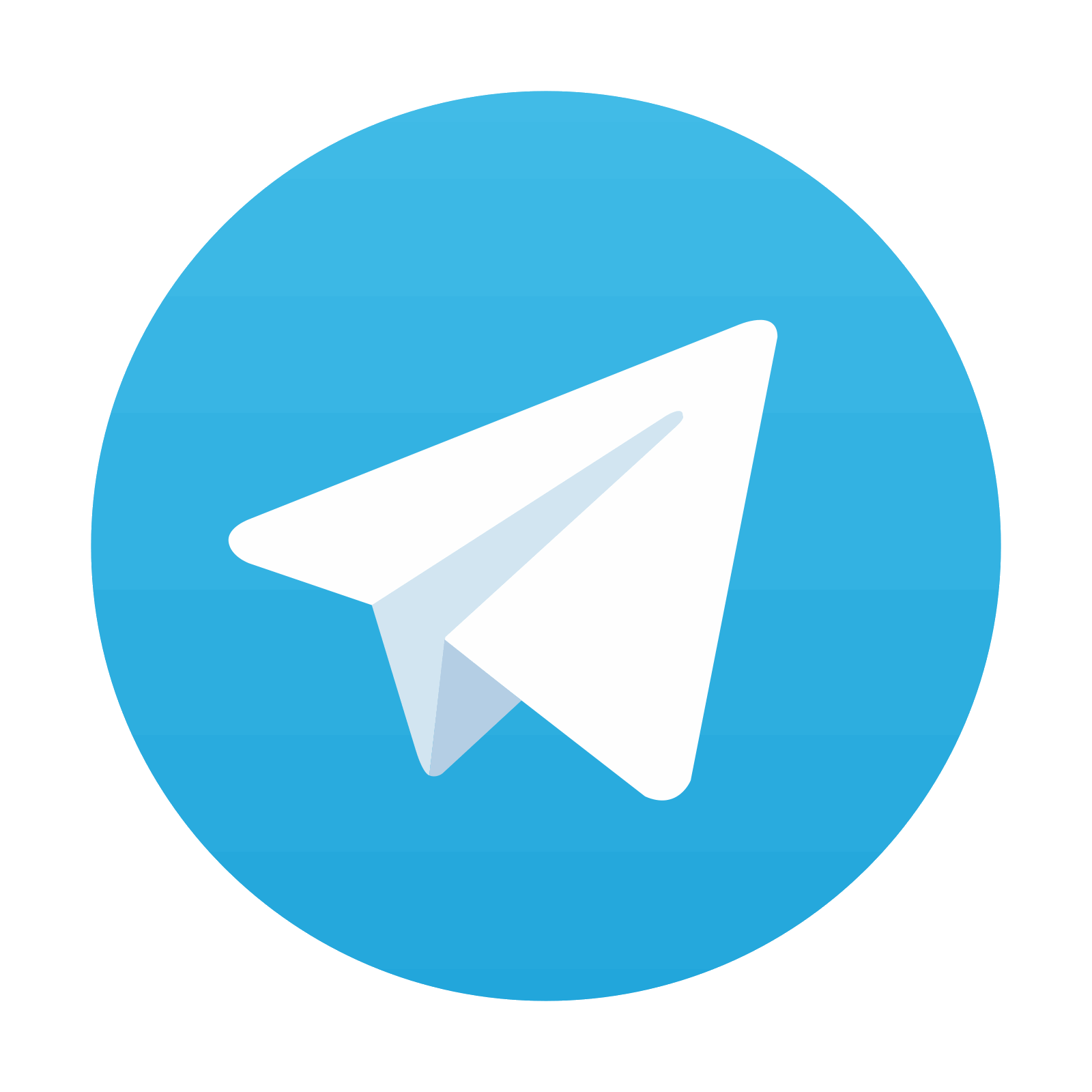
Stay updated, free articles. Join our Telegram channel
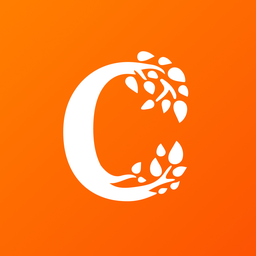
Full access? Get Clinical Tree
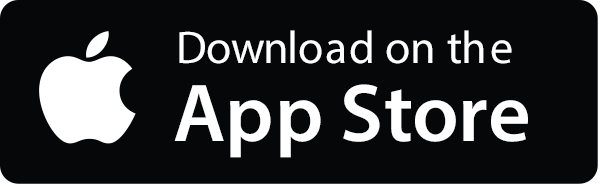
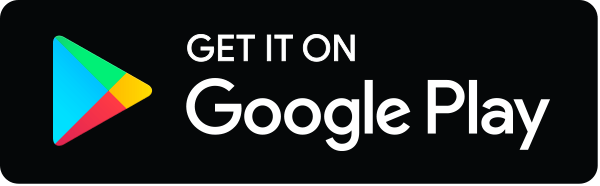