Fig. 1
48-year-old man with prior myocardial infarction in right coronary artery territory. First-pass perfusion MRI (a) and first-pass CT images (b) in short axis view show perfusion deficit consistent with chronic myocardial infarction of the inferior wall of the left ventricle with myocardial wall thinning
2 Dynamic Myocardial CT Perfusion Imaging
Assessment of myocardial perfusion CT requires time-resolved imaging of the myocardial wash-in and wash-out of iodinated contrast material. The utility of myocardial perfusion imaging with MDCT has been established in animal and human trials (Mahnken et al. 2006; George et al. 2006). However, previous CT generations were limited by restricted volume coverage for time-resolved volume image acquisition during the infusion of contrast medium. With the introduction of new generation scanners, including wide detector array CT and dual source CT (DSCT), research on dynamic myocardial CT perfusion imaging is once again in the limelight (George et al. 2009; Dewey et al. 2009; Lell et al. 2009; Leschka et al. 2009). Bamberg et al. investigated the accuracy of CT dynamic stress myocardial perfusion imaging to estimate myocardial blood flow (MBF) in a porcine animal model with variable degrees of induced coronary artery stenosis in comparison with microsphere-derived MBF. The study reported a valid difference but an underestimated correlation of the MBF in CT in comparison with microsphere-derived MBF. Bastarrika et al. investigated the use of adenosine-stress dynamic myocardial volume perfusion imaging with second generation DSCT. In their study, they evaluated the qualitative and quantitative assessment of myocardial blood flow using CT in comparison with stress perfusion and viability MRI (Bastarrika et al. 2010). Their sensitivity, specificity, positive predictive value, and negative predictive value for the detection of myocardial perfusion defects using CT, compared with MRI, were 86.1, 98.2, 93.9, and 95.7 %, respectively, on a per-segment basis. Additionally, their semiquantitative analysis of CT data showed significant differences between ischemic and nonischemic myocardium with a signal intensity upslope that was comparable with MRI-derived values. A similar study by Bamberg et al. concluded that a combined assessment, including coronary artery anatomy evaluation by CT angiography and a dedicated dynamic CT-based stress perfusion imaging to estimate MBF, permits accurate identification of hemodynamically significant coronary artery stenosis (Bamberg et al. 2011). Comparable results were published by Ho et al., who reported a sensitivity, specificity, positive predictive value, and negative predictive value for identifying segments with perfusion defects of 83, 78, 79, and 82 % (Ho et al. 2010). Similar results in studies using DSCT were reported by George et al., using 256- and 320- MDCT (George et al. 2006, 2009).
Despite the promising findings for dynamic myocardial CT perfusion imaging, the modality is still limited by a comparatively high radiation dose. While latest cCTA techniques cut the radiation dose down to 1 mSv, the radiation exposure of a dynamic myocardial CT perfusion examination was reported around 12–13 mSv, which is equivalent to nuclear techniques, e. g., SPECT (Lell et al. 2009; Bamberg et al. 2011). Furthermore, the literature on dynamic myocardial CT perfusion is sparse and more clinical trials are needed before it can be adopted into mainstream clinical use.
3 Delayed Enhancement CT
The presence and transmural extent of an MI are important predictors of functional recovery after surgical and percutaneous revascularization (Selvanayagam et al. 2004). Nowadays, myocardial late enhancement MRI (LE-MRI) is considered the gold standard for the evaluation of the transmural extent of an MI (Kim et al. 1999). Moreover, the phenomenon of myocardial late enhancement (LE) is considered to be an evidence for the presence of nonviable myocardium and is associated with acute as well as chronic MI (Choi et al. 2001; Mahrholdt et al. 2002; Wu et al. 2001). LE-MRI has proven utility in clinical routine for differentiating viable from nonviable myocardium and acute from chronic MI (Abdel-Aty et al. 2004). Myocardial segments exhibiting greater than 50 % transmural extent of delayed enhancement can be defined as nonviable, as 90 % of such segments show no improvement in contractility after revascularization (Kim et al. 2000). With the introduction of MDCT, LE has also become a focus of CT research. On LE-CT examination, a segment affected by MI will show increased attenuation when compared with normal myocardium (Fig. 2). Several animal studies proved the reliability of LE-CT in acute and chronic MI in comparison with MRI, SPECT, and TTC staining (Baks et al. 2006; Buecker et al. 2005; Lardo et al. 2006). Results of these studies not only demonstrate the feasibility of LE-CT, but also a good correlation between the size of an MI in LE-CT and that observed through LE-MRI or TTC staining (Baks et al. 2006; Buecker et al. 2005; Lardo et al. 2006; Mahnken et al. 2007; Brodoefel et al. 2007a). An animal study by Mahnken et al. found that the reduction in infarct size over time is a process that can also be demonstrated using CT (Mahnken et al. 2007). Subsequent human studies published results with sensitivities between 78 and 97 % and specificities from 90 to 98 %, in comparison to SPECT and LE-MRI on a segmental basis (Mahnken et al. 2005; Gerber et al. 2006; Paul et al. 2005). Therefore, CT imaging may aid in predicting clinical outcomes, as the presence and size of both LE and perfusion defects on CT were found to be predictive of myocardial dysfunction after acute MI (Lessick et al. 2007; Sato et al. 2012). In addition to MI size, LE-CT has demonstrated the ability to differentiate between acute and chronic MI. In the acute phase MDCT, myocardial infarction showed higher attenuation values compared to normal myocardium, while “no-reflow” areas revealed hypodense regions surrounded by hyperenhanced myocardium (Buecker et al. 2005). These “no-reflow” areas represent the core of the MI, which can become necrotic as a consequence of intense and constant ischemia. Furthermore, the presence of “no-reflow” areas may be the finding that is most predictive of a residual perfusion defect after revascularization (Paul et al. 2005). In chronic MI, necrotic myocardial cells are replaced by scar tissue in reperfused and occlusive MI presenting with the typical LE. Due to the development of scar tissue with increased interstitial space, the increased distribution volume in the peri-infarction zone might be responsible for the small overestimation of infarct size seen with LE-CT (Wu et al. 2001; Allard et al. 1988).
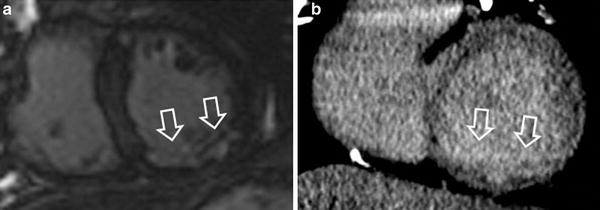
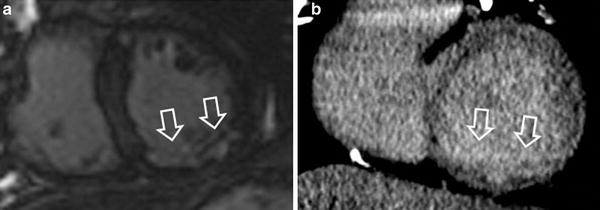
Fig. 2
53-year-old man with prior myocardial infarction in right coronary artery territory. MRI late enhancement (a) and CT late enhancement (b) findings in short axis view are consistent with chronic myocardial infarction of the inferior wall of the left ventricle with myocardial wall thinning
With the introduction of the latest MDCT and DSCT generations, which employ more sophisticated scanning techniques, LE-CT has become a key focus in the area of cardiovascular imaging. The reduction in radiation dose applied during LE-CT must be particularly emphasized. As radiation dose associated with retrospectively ECG-gated CT was reported within the range of 4.5 mSv for an LE-scan, several methods have recently been described to lower the radiation dose (Nieman et al. 2008). An animal study by Broedefel et al. investigated the efficacy of low-dose CT imaging of late enhancement in acute MI. Starting with a standard protocol using 120 kV and 800 mAs for an LE-CT examination, the tube voltage was lowered to 80 kV and the tube current was reduced to 400 mAs; this resulted in higher contrast for late enhancement and good correlation with MRI. Additionally, the lower X-ray tube settings resulted in a radiation dose reduction from 15.6 to 2.8 mSv. (Brodoefel et al. 2007b). Reimann et al. investigated this approach in a feasibility study looking at the practicality of a low dose 80 kV protocol for detecting LE and found promising results (Reimann et al. 2008). In addition to lower tube voltages, the introduction of high-pitch DSCT has permitted further reductions in radiation dose with CT (Lell et al. 2009; Leschka et al. 2009). Using this method for LE-CT, Goetti et al. reported radiation doses of 0.7–1.02 mSv with good accuracy when compared to the gold standard, MRI (Goetti et al. 2011).
Although results from studies using LE-CT to assess myocardial viability have been promising, there are still some points of concern. First, as of this writing, there is no clear agreement on the most appropriate protocol for LE-CT. Two important protocol variables are the scan delay and the mode of contrast administration. The optimal scan delay must allow contrast material to accumulate in infarcted myocardium over several minutes, yet avoid the rapid vanishing of the contrast out of the myocardium. Selection of an ideal scan delay is essential for accurate LE-CT images. Some publications suggest that the best contrast between MI and normal myocardium is seen 5–10 min after injection (Brodoefel et al. 2007b; Deseive et al. 2011).
Although LE-CT does not have a clear place in current clinical practice, it may have a role in dedicated MI imaging in patients with contraindications to MRI. Further research is needed to determine the optimal scan delay and thus improve accuracy. However, current research has shown promising results for radiation dose reduction in LE-CT, which has previously been a major drawback of LE-CT.
4 Dual Energy CT
Dual energy CT (DECT) is one of the latest evolutions in CT technology. Introduced to the market in 2006, DECT is a dual source system equipped with two x-ray tubes and detector arrays mounted in the same gantry, perpendicular to each other (Flohr et al. 2006). The tubes can be operated simultaneously and independently, using different potentials at the same time, generating different X-ray spectra. This has made it possible to scan the same voxel simultaneously with two different energy levels. As described elsewhere, some materials show characteristic levels of CT attenuation (as measured in HUs), depending on the X-ray spectrum to which they are exposed; DECT allows material differentiation in clinical routine, notably the separation of iodine (Johnson et al. 2007). In dual source, single-energy mode, both X-ray tubes are operated with the same potential, usually 120 kV, to achieve a temporal resolution as low as 83 ms. When operated in dual energy mode for cCTA, the tube voltages are typically set to 140 kV on system A and 100 kV on system B (Schwarz et al. 2008). With these DECT settings, the best achievable temporal resolution is 165 ms, which equals that of a 64-slice scanner—a scanner generation that has shown consistently high diagnostic accuracy in the detection of significant coronary artery stenosis compared with cardiac catheterization (Herzog et al. 2007). However, this significant trade-off in temporal resolution comes with the potential benefit of material differentiation using DECT (Johnson et al. 2007). Iodine, which is used as a contrast material in CTA, has a very unique dual-energy characteristic (Zatz 1976). This enables the visualization of iodine distribution in different tissues of the body and the separation demarcation of various materials. For the evaluation of myocardial viability, DECT may be used as a first-pass arterial phase or LE-CT imaging technique (Fig. 3). Recently, Kerl et al. investigated the feasibility and performance of DECT during the arterial phase in cCTA for the detection of chronic infarction, compared with LE-MRI and histopathology, in a porcine model of reperfused myocardial infarction (Kerl et al. 2011a). In this feasibility study, they found a sensitivity and specificity for DECT of 72 and 88 %, respectively, compared to 78 and 92 %, respectively, for LE-MRI, and 60 and 93 %, respectively, for the 100 kV dataset of the DECT scan, all versus histopathology. This comparably low sensitivity of all methods might be explained by the higher heart rate of the piglets, and therefore a reduced image quality as well as motion artifacts. Another study by Zhang et al. found superior results for DECT in terms of sensitivity and specificity (92 and 80 %) for detecting acute myocardial infarction in dogs (Zhang et al. 2010). In the first systematic analysis of the potential usefulness of DECT for comprehensive CAD imaging in humans, rest- DECT correctly identified 26 of 29 (90 %) fixed myocardial perfusion defects observed on SPECT (Ruzsics et al. 2008). Another investigation comparing SPECT and DECT in first-pass arterial phase cCTA, Ruzsics et al. reported an overall sensitivity for DECT of 92 % and a specificity of 93 %, with 93 % accuracy for detecting any type of myocardial perfusion defect seen on SPECT. Contrast defects seen with DECT correctly identified 96 % of 89 fixed and 88 % of 68 reversible myocardial perfusion defects (Ruzsics et al. 2009).
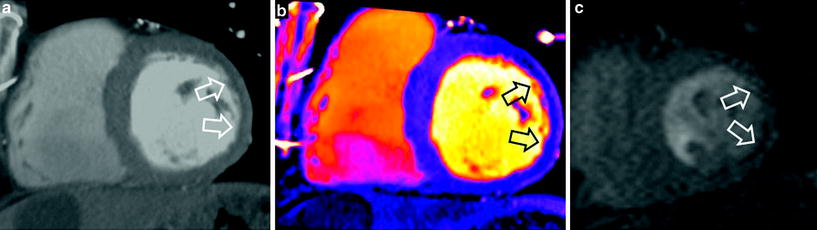
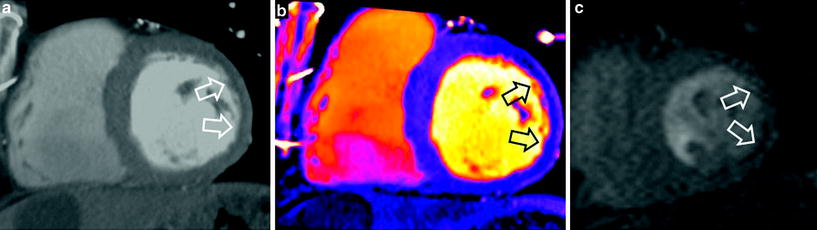
Fig. 3
54-year-old man with prior myocardial infarction in left anterior descending coronary artery territory. First-pass grayscale CT images (a) and DECT color coded perfusion map (b) show lateral hypoenhancement, indicating myocardial infarction, which is in good agreement with findings shown on first-pass perfusion MRI (c)
In summary, there is emerging evidence for the application of DECT first-pass arterial phase imaging as a comprehensive CAD assessment tool. However, further research is required to confirm these results.
In addition to DECT first-pass arterial phase imaging, recent research investigated the use of LE-DECT for imaging MI and viability (Fig 4). Deseive et al. studied the performance of LE-DECT, in comparison with LE-MRI, for the detection of irreversibly damaged myocardium in a porcine model of reperfused chronic myocardial infarction using histopathology as the standard of reference (Deseive et al. 2011). They reported a sensitivity of 76 % and a specificity of 93 % for LE-DECT compared to a sensitivity of 62 % and sensitivity of 97 % in 100 kV imaging. These results are concordant with the results of Bauer et al. (2010), who investigated DECT-LE in humans matched with delayed LE-MRI. In this study, 36 patients with coronary artery bypass grafts were examined with LE-DECT and 3-T MRI, resulting in a sensitivity of 70 % for LE-DECT. However, only 22 of the patients (61 %) showed delayed enhancement on MRI. Again, in this patient population, the DECT studies suffered from artifacts arising from sternal wires and implanted metallic devices, affecting sensitivity. Apart from assessing MI extent and myocardial viability, DECT has an additional benefit concerning the reduction of radiation dose during cCTA (Kerl et al. 2011b).
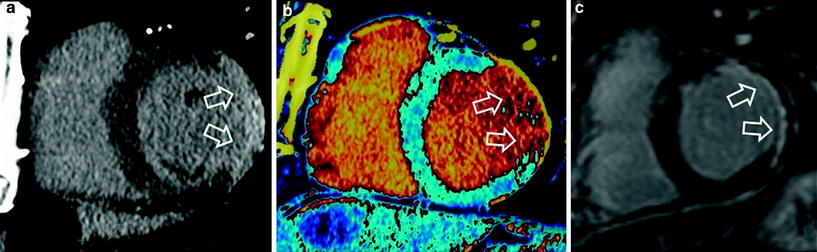
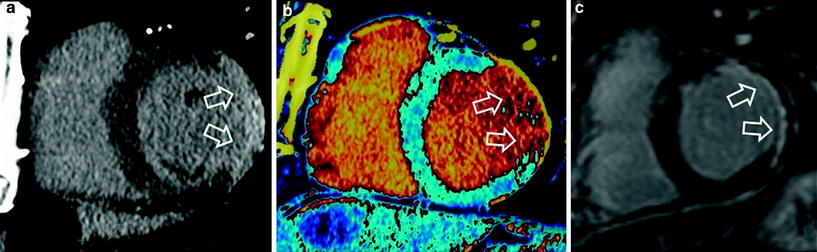
Fig. 4
54-year-old man with prior myocardial infarction in left anterior descending coronary artery territory. Late enhancement grayscale CT images (a) and late enhancement DECT color coded perfusion map (b) show lateral hyper enhancement, indicating myocardial infarction, which is in good agreement with findings shown on late enhancement MRI (c)
In a nutshell, DECT shows promising results in arterial phase cCTA as well as in LE-CT. However, DECT imaging of the myocardium needs further refinement to reduce motion and metal artifacts before widespread use can be expected. If advanced artifact reduction can be achieved, DECT may become a multipurpose method for comprehensive CAD imaging and sophisticated myocardial viability examination.
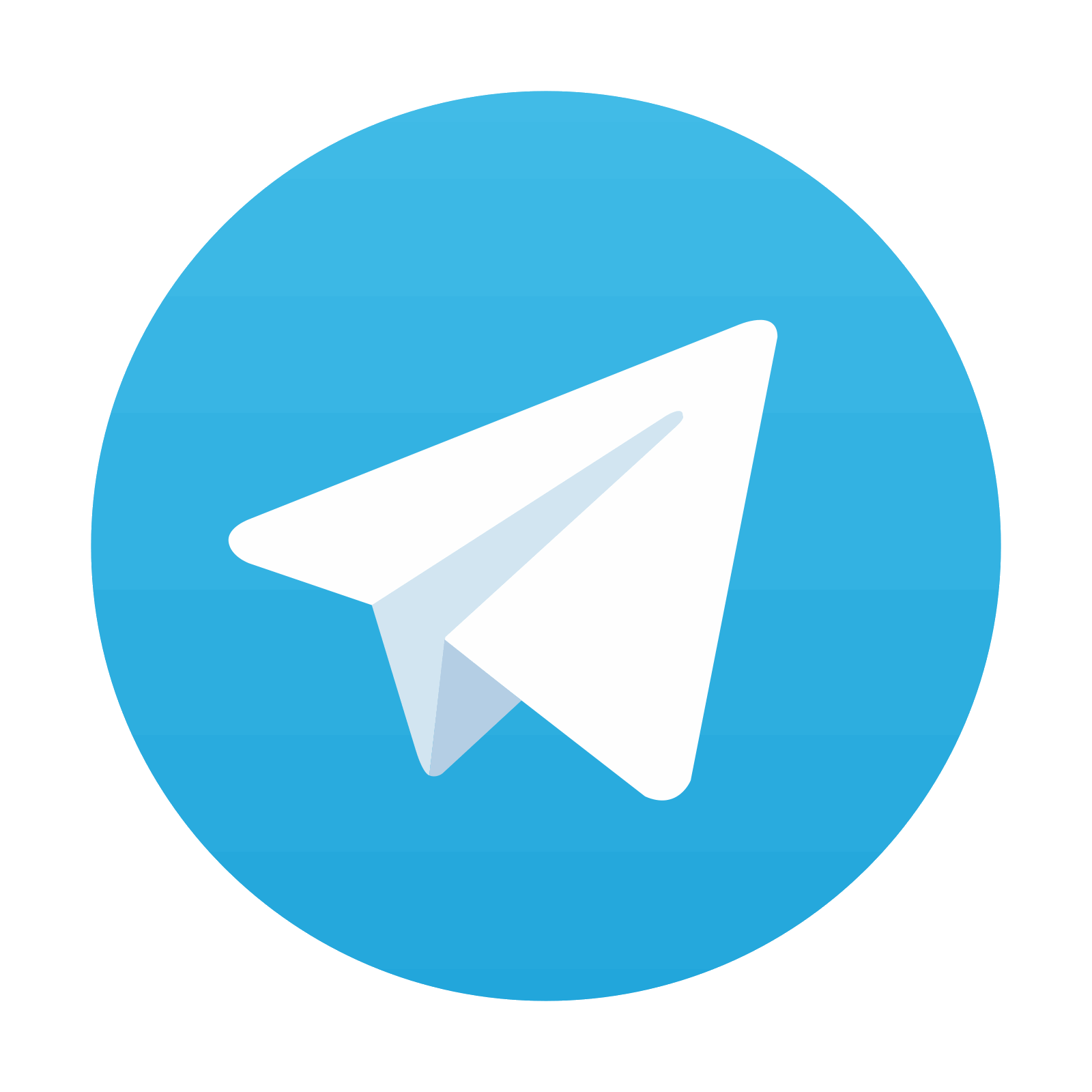
Stay updated, free articles. Join our Telegram channel
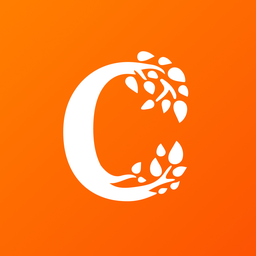
Full access? Get Clinical Tree
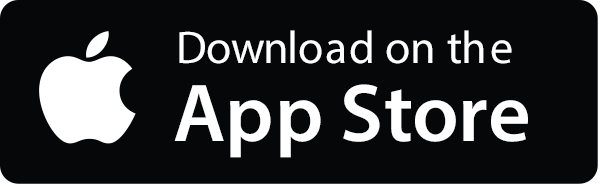
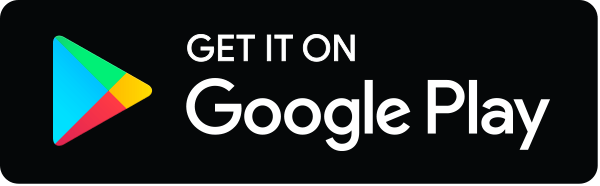