Plaque characteristic
MRI
CT
US
PET
SPECT
DSA
Active inflammation
✓
x
x
✓
x
x
Thin FC with large LRNC
✓
✓
x
x
x
x
FC disruption
✓
x
x
x
✓
x
Severe stenosis
✓
✓
✓
x
x
✓
Intra-plaque haemorrhage
✓
x
x
x
x
x
Expansive remodelling
✓
x
✓
x
x
x
Superficial calcified nodules
✓
✓
x
x
x
x
Yellow colouring on angioscopy
x
x
x
x
x
x
Endothelial dysfunction
✓
x
x
✓
x
x
Fibrous Cap
The atherosclerotic plaque is covered by a fibrous cap, which consists of a mixture of endothelial cells, collagen fibres and matrix glycoproteins overlying the lipid-rich core (Fig. 1) [19]. A thin fibrous cap has been shown to be associated with higher risks of plaque rupture [10, 11, 20, 21]. Thin or ruptured fibrous cap detected using carotid MRI, in turn, is correlated with ipsilateral ischaemic event [22]. Using a three-dimensional multiple overlapping thin-slab angiography protocol, one group categorised fibrous cap into (1) intact and thick, (2) intact and thin and (3) ruptured, and demonstrated that these findings correlated closely (89 %) with histological assessment [23]. Multi-sequence, high-resolution MRI used to quantify fibrous cap and lipid core thickness also showed strong agreement with histological results, and the fibrous cap-to-lipid core thickness ratio was suggested as a method for characterising vulnerable plaques in vivo [24]. Similarly, in vivo high-resolution contrast-enhanced MRI (CEMRI) was found to provide moderate-to-good measurements of the length and area of intact fibrous cap and lipid-rich necrotic core [25]. In a study of asymptomatic patients with <50 % carotid stenosis, men were shown to have a higher level of thin or ruptured fibrous cap and a larger percentage volume of lipid-rich necrotic core, as compared to women, reflecting the higher risk of subsequent stroke in the former [26]. Treatment of carotid stenosis patients with an anti-platelet agent, cilostazol, was found to significantly increase the percentage of fibrous component in a plaque while simultaneously decreasing the percentage of lipid and haemorrhagic components [27]. These studies provided good evidence that MR imaging of atherosclerotic plaques offers a non-invasive but accurate means of quantifying the fibrous cap.
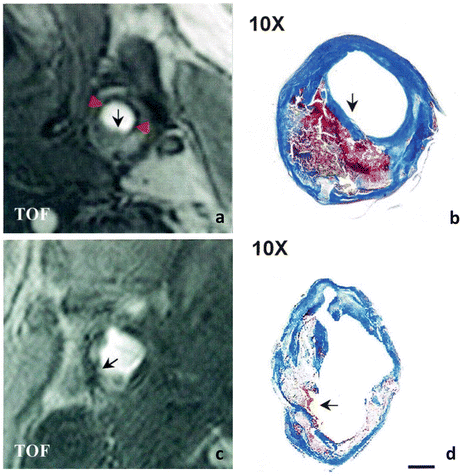
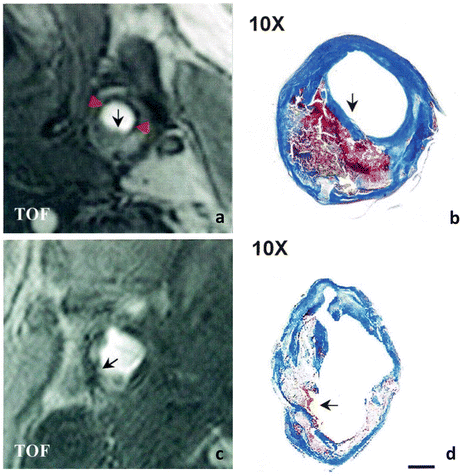
Fig. 1
Comparison of carotid atherosclerotic plaques with intact and ruptured fibrous caps. MRI TOF image of an atherosclerotic plaque with intact fibrous cap (a) shows a hypointense band (red arrowheads) lining most of the luminal surface except over the bulk of the plaque (black arrow). TOF image of a heavily calcified plaque with ruptured fibrous cap (c) shows a discontinuous hypointense band surrounding the lumen (arrow). Mallory’s trichrome-stained sections of the corresponding plaques (b and d) show a large necrotic core with overlying intact (b) or ruptured (d) fibrous cap (arrow) (scale bar 1 mm). Adapted from Yuan et al. (2002) Circulation 105:181–185
Disruption of the fibrous cap, e.g. rupture or ulceration, is often seen in histopathological specimens from patients with previous TIA or stroke [28, 29]. This is mainly caused by the pulsatile force imposed by the arterial blood on the atherosclerotic plaque during the cardiac cycle, which leads to fibrous cap fatigue, and subsequent rupture [30]. High-resolution MRI was used to identify ruptured fibrous cap, which was shown to be associated with recent TIA or stroke [16]. In patients who had suffered a stroke, the presence of a thin or ruptured fibrous cap is associated with higher risk of recurrence [31]. Hatsukami et al. successfully used MRI to detect ruptured plaques to a high degree of consistency with histological findings [23]. Multi-sequence cross-sectional MRI has been used to detect ulceration of carotid atherosclerotic plaques [32, 33]. This technique can be further improved by the addition of longitudinal black-blood MR angiography which enhances the ability to identify plaque ulceration [34]. Nevertheless, it must be borne in mind that the spatial resolution of MRI is lower than the histological definition of a thin fibrous cap, thus limiting its ability to accurately determine the thickness of these caps [25].
Lipid-Rich Necrotic Core
The size of the lipid-rich necrotic core of an atherosclerotic plaque can also determine its vulnerability. This is believed to be due to the combined effect of hypoxia and necrosis in the lipid core and the accompanying neovascularisation, which promote intra-plaque haemorrhage, which itself is a contributor to the development of vulnerable plaque. The size of lipid-rich necrotic core and % wall volume are plaque characteristics associated with cerebral infarction [35]. A multicentre cross-sectional study using multi-contrast MRI identified the maximum proportion of the arterial wall occupied by the lipid-rich necrotic core as the strongest predictor of intra-plaque haemorrhage [36]. This has potential to be used as a risk-stratifying strategy. Meanwhile, Young et al. performed echo-planar diffusion-weighted imaging sequence along with standard MRI to generate apparent diffusion coefficient (ADC) maps for patients with confirmed moderate-to-severe carotid artery stenosis. They found that quantitative ADC values correlated well with histological examination, with heavier lipid staining corresponding to low ADC values [37]. In addition, they also showed that DWI can be used to differentiate lipid-rich necrotic core from fibrous cap [37]. A recent prospective study calculated the carotid atherosclerosis score (CAS) based on maximal wall thickness and % lipid-rich necrotic core, and found a significant increasing relationship with disrupted luminal surface and plaque progression, features characteristic of a vulnerable plaque [38].
Multi-contrast MRI has also been utilised as an outcome measure for a study investigating the effect of lipid-lowering therapy on carotid atherosclerotic plaque [39]. By following up patients on atorvastatin monotherapy or in combination with other lipid-lowering drugs annually, the authors demonstrated that intensive lipid-lowering therapy significantly decreased the volume and percentage of arterial wall occupied by lipid-rich necrotic core. This lipid-depleting effect was observed after 1 year of treatment and preceded the regression of the plaque itself [39]. Sun et al., meanwhile, showed that short-term (mean 6.9 months) changes in the characteristics or composition of lipid-rich necrotic core can be followed up using serial MRI of the carotid artery, and demonstrated that the progression of lipid-rich necrotic core can be affected by intra-plaque haemorrhage [40].
Vessel Wall Thickness
Arteries may also undergo expansive remodelling, whereby atherosclerotic plaques progress by pushing the vessel wall outwards, rather than causing narrowing of the lumen, making it difficult to be identified using methods such as DSA [7]. A study looking at the impact of simvastatin showed that the lipid-lowering therapy significantly decreased vessel wall thickness and vessel wall area at 12 months, 18 months and 24 months, as assessed by black-blood fast spin-echo MRI [41, 42]. However, further investigations revealed that this effect was not dependent on the dose of the simvastatin used, with both high-dose (80 mg/day) and low-dose (20 mg/day) patients demonstrating similar improvements in vessel wall thickness and area [43]. Another group compared the utility of MRI-derived plaque volume and two-dimensional ultrasound intima-media thickness (IMT) of the carotid plaque in patients on 6-month statin therapy. They found that while IMT did not detect any changes, measurements from MRI showed a significant reduction in plaque volume, highlighting the advantages of MRI over ultrasound in assessing the response to treatment in patients with atherosclerotic diseases [44].
A recent phase 2b, multicentre clinical trial investigating the effect of dalcetrapib on atherosclerosis used multiple non-invasive imaging modalities, including MRI, CT and PET, as its primary end points looking at vessel structure and inflammation [45]. MRI was used to assess vessel area, wall area, wall thickness and normalised wall index after 24 months of treatment. It was found that MRI-derived change in total vessel area was reduced in patients who were given dalcetrapib, who also did not show progression of carotid plaques [45]. A prospective cohort study on the natural history of carotid and femoral atherosclerosis also employed high-resolution MRI as its non-invasive tool assessing lumen area, vessel area and vessel wall area [46]. The authors reported a progressive decrease in lumen area accompanied by an increased vessel wall area, while the vessel area remained unchanged [46].
Intra-plaque Haemorrhage
The chronic inflammatory process taking place within an atherosclerotic plaque can lead to neovascularisation. These newly formed vessels tend to be weak and fragile, and may rupture easily leading to haemorrhage into the plaque. Recurrent intra-plaque haemorrhage then further perpetuates the inflammatory process, resulting in a vicious cycle. The ability of MRI to detect intra-plaque haemorrhage depends on the degradation of haemorrhage into hemosiderin and ferritin, two major iron-storing proteins in the bloodstream. Haemorrhage appears as a high signal on T1-weighted sequences and low signal on T2-weighted sequences.
The prevalence of intra-plaque haemorrhage as identified by MRI of the carotid artery was significantly higher in symptomatic, compared to asymptomatic, patients [47]. MRI-defined intra-plaque haemorrhage is a strong independent predictor of recurrent ischaemic events and strokes in symptomatic patients with significant carotid stenosis (>50 %) [48]. Atherosclerotic plaques undergo a significantly higher progression rate following the development of intra-plaque haemorrhage, suggesting that early identification of intra-plaque haemorrhage may be clinically useful in ensuring optimal management of patients [49]. Following a stroke, patients with MRI-detected intra-plaque haemorrhage have a higher risk of suffering from a recurrence [31]. Using magnetisation transfer (MT) magnetic resonance (MR) to calculate the magnetisation transfer ratio (MTR), Qiao et al. were able to distinguish low-protein areas from high-protein areas within a plaque, which has different MTRs. Furthermore, they showed that old and recent intra-plaque haemorrhages displayed higher MTR compared to fresh haemorrhage, providing a novel avenue for detecting these vulnerable plaques [50]. The location of haemorrhage can also be identified more precisely with a high-resolution MRI, in distinguishing intra-plaque haemorrhage from juxtaluminal haemorrhage [51]. As mentioned earlier, treatment with an anti-platelet agent cilostazol significantly reduced the percent area occupied by the haemorrhagic component within a carotid atherosclerotic plaque [27]. In patients with severe carotid stenosis (>50 %) who are also symptomatic, MRI-detected plaque haemorrhage occurs less frequently in females compared to males [52].
Improvements in MRI technology also helped to promote the feasibility of identifying intra-plaque haemorrhage. Magnetisation-prepared rapid acquisition gradient-echo (RAGE) was shown to be highly sensitive and specific in detecting intra-plaque haemorrhage, with a high signal-to-noise ratio [53]. One group developed a novel slab-selective phase-sensitive inversion-recovery (SPI) technique which demonstrated a superior ability to detect intra-plaque haemorrhage, with significantly improved intra-plaque haemorrhage-wall contrast-to-noise ratio and blood suppression efficiency compared to traditional methods [54].
Inflammation
Atherosclerosis is essentially a chronic inflammatory process affecting the tunica intima of the arteries [55]. There is an influx of inflammatory cells including macrophages, neutrophils, T lymphocytes and B lymphocytes into the intima, along with the production and release of a variety of pro-inflammatory factors [55, 56]. This, coupled with the associated neovascularisation, is implicated in the development of vulnerable plaques. Therapies targeting the inflammatory process are also beginning to be used in the treatment of atherosclerosis.
The accumulation of activated macrophages indicates areas of active inflammation. MRI can be utilised to identify these areas, which in turn may suggest increased vulnerability of an atherosclerotic plaque [57, 58]. One technique that has been used is ultra-small superparamagnetic iron oxide (USPIO)-enhanced MRI, in which the USPIO acts as a non-gadolinium-based contrast agent that is selectively taken up by activated macrophages in areas of inflammation. Accumulation of USPIO in macrophages was clearly detected in almost all (10/11) of the patients in one in vivo study, which correlated with ruptured or rupture-prone atherosclerotic plaques [59]. Trivedi et al. subsequently characterised the time frame of USPIO-enhanced MRI signal, demonstrating that the optimal window for detection was 24–36 h post-infusion of contrast (Fig. 2) [60]. USPIO-enhanced MRI of the carotid artery has also been tested as an outcome measure for the effect of lipid-lowering agents on atherosclerotic plaques in a clinical trial (ATHEROMA). The authors showed that treating patients with known carotid plaques with high-dose (80 mg/day) atorvastatin significantly reduced USPIO-defined inflammation, from as early as 6 weeks after starting treatment [61]. This highlights the potential of USPIO-enhanced MRI as a novel imaging modality in assessing plaque vulnerability and the effectiveness of medical therapies.
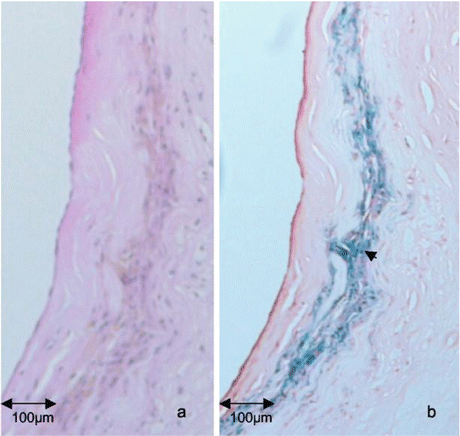
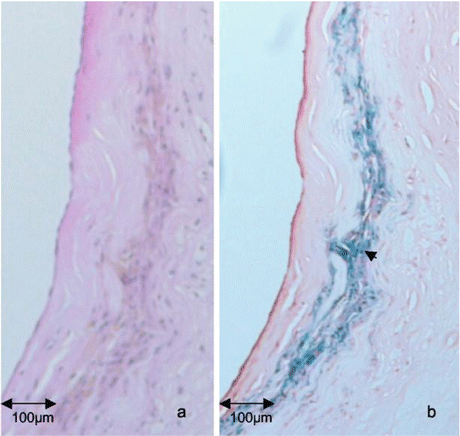
Fig. 2
Co-localisation of USPIO with areas of high macrophage content in the fibrous cap of an atherosclerotic plaque. H&E (a) and Perls staining (b) on a section of atherosclerotic plaque shows areas of USPIO accumulation co-localising with areas of high macrophage content in the fibrous cap region at high power (arrow). Adapted from Trivedi et al. (2004) Stroke 35:1631–1635
A further study demonstrated an association between USPIO-enhanced MRI signal and biomechanical stress, suggesting a complex interaction between different risk factors in contributing to the vulnerability of atherosclerotic plaques [62]. On the other hand, there appears to be no correlation between inflammation as detected by USPIO-enhanced signal change and luminal stenosis [63]. However, plaques that have undergone intra-plaque haemorrhage also had higher levels of macrophage and lymphoid cells, suggesting a link between intra-plaque haemorrhage and inflammation [64].
Another feature of inflammation in atherosclerosis is neovascularisation, whereby new, weak and fragile vessels encroach into the areas affected, increasing the risk of intra-plaque haemorrhage. A direct correlation exists between the extent of microvasculature within a plaque and the risk of intra-plaque haemorrhage and subsequent rupture [65, 66]. To visualise these new vessel formation in atherosclerotic plaques, dynamic contrast-enhanced MRI (DCE-MRI), a technique originally developed for neuro-oncology is beginning to be used. DCE-MRI of the human carotid arteries demonstrated a hyperintense outer rim surrounding the vessel, indicating angiogenesis of the wall itself, which was thicker in older patients [67]. DCE-MR angiography, meanwhile, provided a highly accurate image of the areas around the atherosclerotic plaque, including plaque morphology and the thickened arterial wall [68].
Kinetic modelling is used to analyse the images obtained, providing information about the different tissues imaged. In the case of microvasculature formation around the atherosclerotic plaque, two main parameters are used, i.e. fractional plasma volume (V p) and transfer constant of the contrast agent (K trans). They represent the intravascular and extravascular spaces, respectively. V p correlates well with histological findings of neovascularisation, and is believed to represent the actual microvascular volume [69, 70]. In contrast, K trans reflects the permeability of the microvasculature, and correlates with the accumulation of macrophages within the plaque [70].
MRI contrast agents generally elicit a non-specific signal enhancement in tissues. Major studies are under way to discover more specific markers which can accurately identify lipid components [28, 71], thrombus [72], inflammatory mediators [73–75] and apoptotic cells [76]. A study using gadolinium-enhanced MRI on carotid plaque revealed an association between contrast enhancement and characteristics of a vulnerable plaque, including neovascularisation and macrophage infiltration [77].
Biomechanical Stress
Local mechanical loading can lead to the formation of intraluminal thrombus as well as plaque haemorrhage. This is mainly due to an increased stretch force causing the rupture of neovessels, resulting in the formation of intra-plaque haemorrhage [78]. Arteries under constant biomechanical stress due to hyperkinetic or turbulent flow, such as those undergoing chronic inflammation from atherosclerosis, have a higher tendency to rupture [79]. Arteries with high stiffness as measured by aortic pulse wave velocity were associated with the presence of an atherosclerotic plaque, as well as the characteristic features of a vulnerable plaque such as intra-plaque haemorrhage [80].
Significant differences in plaque stress were observed between the diseased and non-diseased segments of the artery [81]. Similarly, carotid plaques in symptomatic patients, especially those suffering from recurrent TIAs, tend to be under a higher biomechanical stress, when compared to asymptomatic ones (Fig. 3) [81]. High-resolution MRI performed on stroke or TIA patients showed that haemorrhagic plaques tended to have higher biomechanical stresses than non-haemorrhagic ones [82, 83]. Measurements of arterial distensibility have also been used to detect carotid stenosis (>15 %) resulting from atherosclerotic plaques [84]. In this study, significantly higher distensibility was detected in the common segment of the carotid artery compared to the internal carotid segment in healthy patients, whereas no segmental differences in distensibility were seen in the disease population [84].
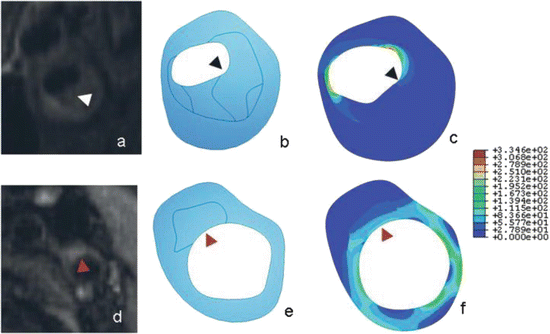
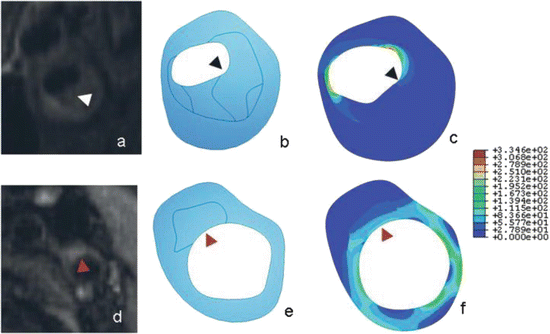
Fig. 3
Comparison of stress maps of atherosclerotic plaques between symptomatic (a–c) and asymptomatic individuals (d–f). Magnetic resonance images (a and d), geometry delineations (b and e) and stress maps (c and f) for symptomatic (a–c) and asymptomatic (d–f) individuals. The symptomatic plaque (a–c) is more stenotic and has a large lipid core (arrowhead), with higher peak stresses in distinct foci while the asymptomatic plaque (d–f) is predominantly fibrous (arrowhead). Adapted from Trivedi et al. (2007) J Neurosurg 107:536–542
Conclusion
Since the shift of focus on the pathophysiology of atherosclerosis from luminal stenosis to the characteristics of carotid plaque, much effort has been put in to find ways of elucidating the plaque components non-invasively. Features associated with a vulnerable plaque, i.e. one at a high risk of resulting in an ischaemic event, such as thin fibrous cap, large volume of lipid-rich necrotic core, intra-plaque haemorrhage and active inflammation, have all been effectively imaged using MRI. However, many of those experiments were only at a preliminary stage, and further studies involving larger populations are warranted. With further validation and additional supporting evidence, it is likely that MRI will become the frontline investigative tool for imaging carotid atherosclerotic plaques, including for diagnosis, surveillance and follow-up post-treatment.
References
1.
2.
3.
Rothwell PM, Eliasziw M, Gutnikov SA, Fox AJ, Taylor DW, Mayberg MR, Warlow CP, Barnett HJ. Analysis of pooled data from the randomised controlled trials of endarterectomy for symptomatic carotid stenosis. Lancet. 2003;361:107–16.CrossRefPubMed
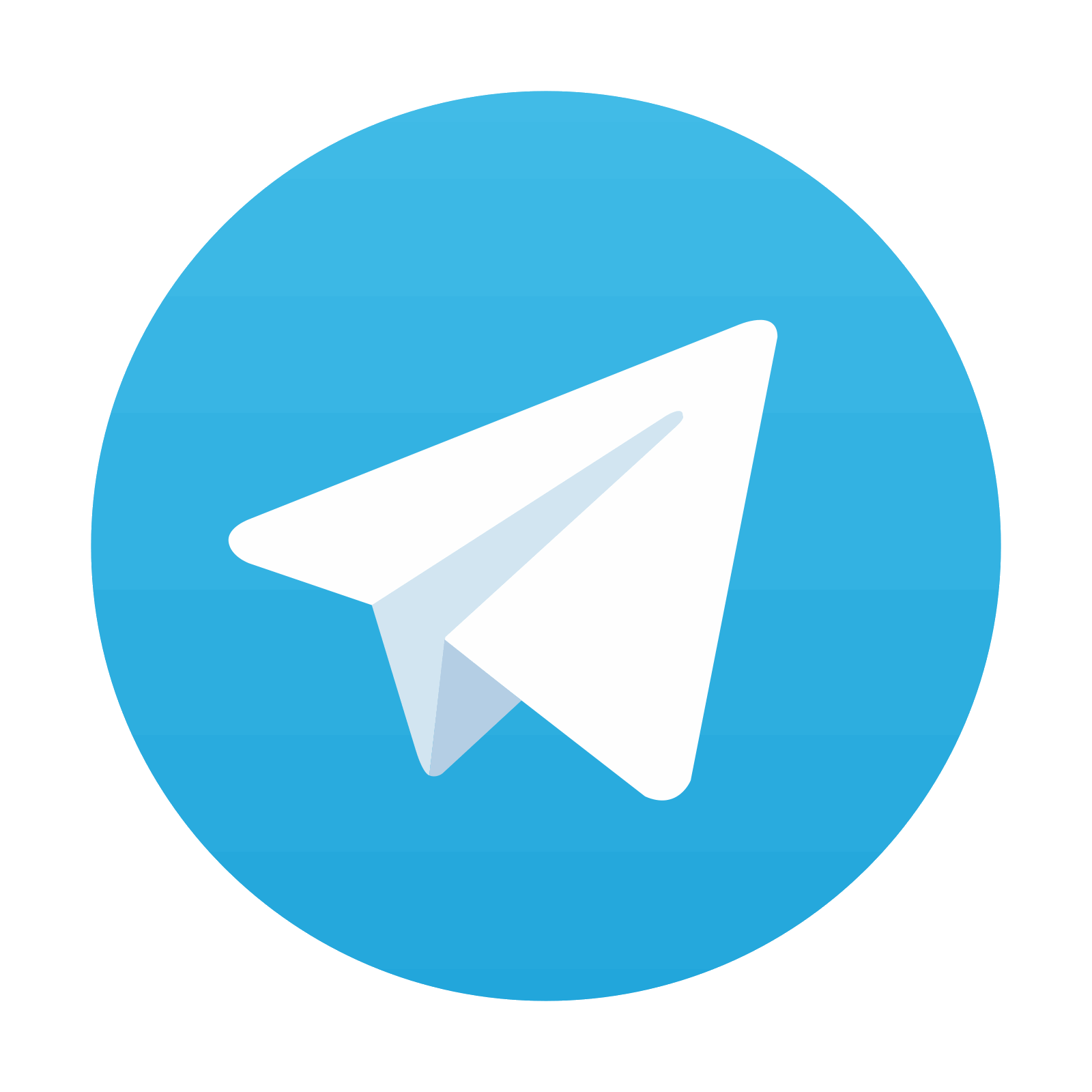
Stay updated, free articles. Join our Telegram channel
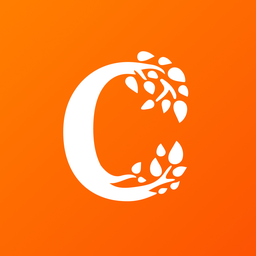
Full access? Get Clinical Tree
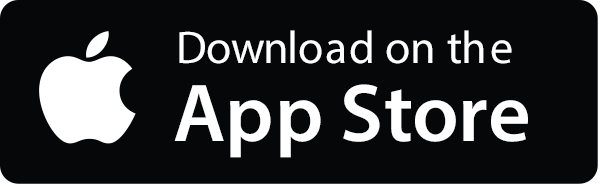
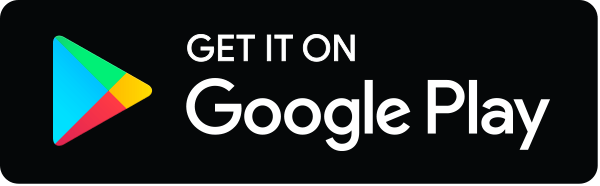