Fig. 2.1
These (a) anterior-posterior (AP) and (b) lateral femur radiographs of a patient with osteosarcoma reveal features typical of a malignant bone tumor including Codman triangles (arrows) and sunburst periosteal reaction (curved arrows)
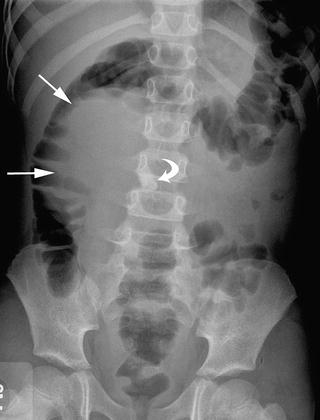
Fig. 2.2
This AP abdominal radiograph of a girl with right ovarian teratoma reveals mass effect on the right colon (arrows) and a toothlike abdominal calcification (curved arrow), features suggestive of this diagnosis
Ultrasonography
Medical ultrasonography (US) utilizes handheld transducers that are placed on the body surface and emit and receive sound waves ranging from 2 to 20 MHz. Higher frequencies provide higher resolution images but have only limited tissue penetration while lower frequency waves penetrate deeper but provide less image resolution. Modern broadband ultrasound transducers are designed to allow the operator to adjust the emitted sound wave frequency for visualization of a structure of interest. In general, frequencies in the range of 8–20 MHz image structures near the transducer and frequencies in the 2–6 MHz range image deeper structures [5, 6]. Tissue harmonic imaging and pulse inversion harmonic imaging allow the transducer to receive both the fundamental transmitted frequency and its harmonic frequencies and have become standard in many applications. These techniques increase the contrast of lesions while reducing the effect of some artifacts, but are limited to shallower depths [6].
Children are ideal candidates for US because their small body habitus, relative to adults, allows placement of the US transducer near the structure of interest, thus reducing signal attenuation. Ultrasound has numerous other advantages that make it particularly useful in the pediatric population. Perhaps most importantly it does not expose the patient to the potential harmful effects of radiation, a topic of considerable concern in this age group. It has the added benefits of being portable, does not require sedation, has Doppler capabilities to dynamically assess vascularity, allows real-time visualization of the movement of abdominal structures relative to each other and is less costly than CT and MRI. Additionally, US is usually readily available and does not require pre-procedure preparation. Drawbacks of US are that it is operator dependant, lacks image resolution compared to CT and MRI, is limited by artifact caused by bowel gas, and it can be difficult to visualize deep-seated structures in obese or adult-size patients.
Despite these potential limitations US remains the modality of choice for the initial assessment of a suspected abdominal mass in children and can provide important clues to the diagnosis. For example, real-time assessment of a right upper quadrant mass allows visualization of the mass relative to the liver and kidney during respiration. During continuous dynamic US imaging, masses separate from these solid organs move independently during breathing whereas masses arising within them move in union with their organ of origin. Ultrasound can reveal the solid or cystic nature of a mass, information that can be extremely useful. Cystic structures appear anechoic or sonolucent on US while solid tissue appears echogenic (Fig. 2.3). Regarding liver masses, US can reveal whether the tumor is solitary or multifocal as is seen with multifocal or diffuse hepatic hemangiomas of infancy, metastatic neuroblastoma, and multifocal hepatoblastoma [7]. Ultrasound can detect calcifications within tumor which appear as bright echogenic foci with posterior shadowing. This finding might suggest an ovarian teratoma when seen in association with an abdominal or pelvic mass in an adolescent girl or neuroblastoma when associated with a retroperitoneal mass in a young child [8]. With the use of high-resolution transducers, US is ideal for assessment of superficial structures such as the thyroid, the scrotum, the eyes, and soft-tissue masses in the head, neck, or extremities (Fig. 2.4). Taken together with the clinical presentation and age of the patient, US imaging features of abnormalities of these structures can often provide a specific diagnosis or greatly narrow the differential diagnosis [9–12].
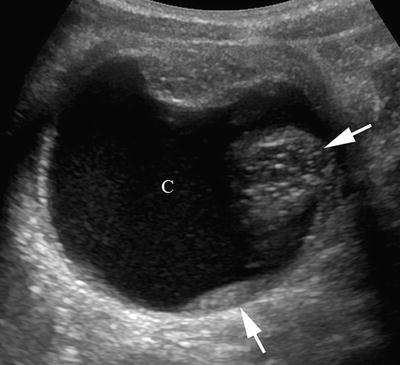
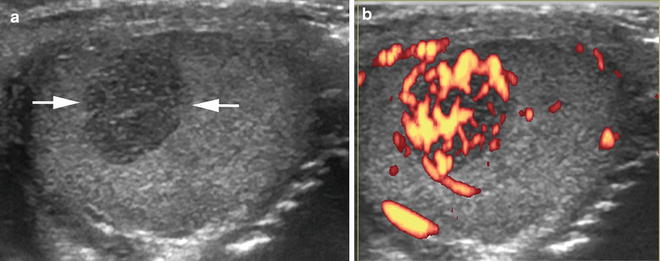
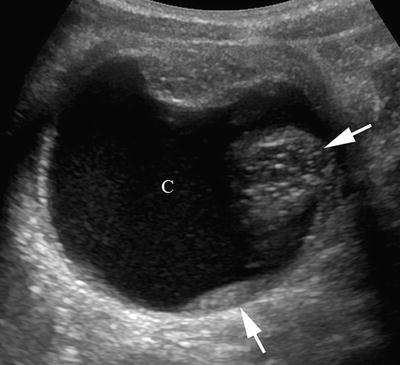
Fig. 2.3
This transverse ultrasound (US) image of an ovarian teratoma reveals the cystic (C) and solid (arrows) components that are typical of this tumor
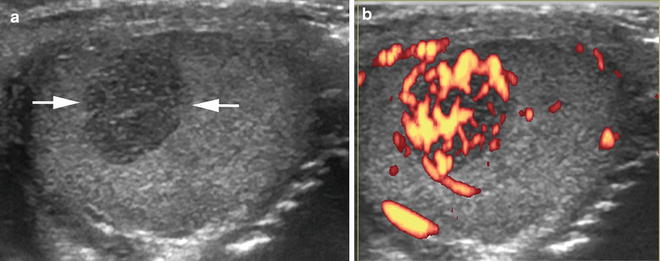
Fig. 2.4
This (a) transverse US image of the testicle reveals a well-defined, small, solid, hypoechoic mass (arrows) that on (b) power Doppler imaging appears hypervascular (orange structures) in this boy with a testicular Sertoli-Leydig cell tumor
Doppler evaluation has numerous applications in the assessment of pediatric tumors. It can show increased blood flow and disorganized vasculature in tumors such as in testicular lymphoma or primary gonadal germ cell tumors [12]. Doppler of hepatic hemangiomas typically shows both arterial and venous waveforms with minimal systolic-diastolic variation in contrast to primary liver malignancies that show high-velocity blood flow [7]. Doppler is also useful in detecting vascular invasion, such as invasion of the renal vein and inferior vena cava by Wilms tumor or the hepatic or portal veins by hepatoblastoma [7, 13].
Because it does not involve ionizing radiation and does not require sedation, ultrasound is particularly appealing for screening patients with syndromes that predispose them to developing abdominal tumors. For example, patients with Beckwith-Wiedemann, Denys-Drash, WAGR (Wilms tumor, aniridia, genitourinary anomalies, mental retardation), Fanconi anemia, and several other syndromes have a risk of developing Wilms tumor (WT). However, the utility of US screening in these patients remains controversial [14]. Due to the rarity of these syndromes no randomized trials have been performed to compare the outcome of screened versus unscreened patients. Furthermore, because survival of patients with WT is greater than 90 % for those with localized disease and over 70 % for those with metastatic disease the benefit of early detection is debatable. Regardless, current efforts are aimed at identifying patients at the earlier and more treatable stages of disease [15, 16]. While there is no physical harm from sonography the emotional stress caused by vigilant screening should not be ignored. Current recommendations for screening children with various syndromes that predispose them to Wilms tumor have recently been published. The kidneys should be imaged with a high-resolution transducer; generally a 7–10 MHz transducer for infants and 6–8 MHz transducer for toddlers. When suspicious lesions are identified the imaging should be repeated within 1 week at a specialist center [14]. Children with other cancer predisposition syndromes also benefit from abdominal screening US to detect associated tumors in other organs. This subject is beyond the scope of this chapter but has been recently reviewed [17].
In the pediatric oncology setting US is valuable for guiding biopsy of newly diagnosed masses. In experienced hands US can safely be used to guide biopsy of a wide variety of tumors including rhabdomyosarcoma, non-rhabdomyosarcoma, soft-tissue sarcomas, neuroblastoma, hepatoblastoma, peripheral pulmonary lesions, and even anterior mediastinal masses requiring core biopsy [18–23]. Ultrasound guidance has the advantage of allowing real-time assessment of tumor vascularity and the relationship of the tumor to major vessels. This information can alert the interventional radiologist to the potential for post-procedural hemorrhage and help direct biopsy away from vascular structures. Additionally, because US machines are portable they can be used as a secondary guidance device in conjunction with CT or fluoroscopically directed biopsies [18, 23].
Computed Tomography
In pediatric oncology, cross-sectional imaging modalities (CT and MRI) are essential tools in patient management. These modalities provide valuable information for formulating a differential diagnosis, staging the tumor, monitoring treatment response, and detecting recurrences. However, with regard to CT, it is important to consider that the developing tissues of pediatric patients are more sensitive to the harmful effects of ionizing radiation than those of adults. Additionally, relative to adults, children have a longer life-span in which to develop adverse sequelae of radiation exposure, which can occur decades later [24]. The pediatric radiologist must have knowledge of the proper use of these imaging modalities so that they can assist oncologists, surgeons, and radiation oncologists in developing rational and appropriate imaging guidelines for therapeutic protocols.
Since the sentinel article by Pierce and Preston in 2000 describing the effects of low-dose radiation exposure [25], there has been an increasing awareness of the detrimental effects of ionizing radiation in children, especially from CT scans. Since then significant progress has been made in reducing the number of CT scans performed, particularly in the pediatric emergency setting. Advances have also been made in optimizing the scanning technique to reduce radiation dose while maintaining image quality [26–28]. However, children with cancer are particularly at risk because they undergo repeated exposure to radiation and the effects are cumulative over time. Modern cancer therapies have resulted in an overall survival rate of 83 % in children [29] and, therefore, the long-term effects of cancer therapy, including radiation exposure, are now being fully realized. Late effects from cancer therapy are becoming the driving force in tailoring pediatric cancer therapies and challenge the radiologist to apply the ALARA principle whenever possible.
The primary ways to minimize radiation exposure from CT are to require justification for the scan being done and optimization of the technique. In general, a CT is not indicated if the same information can be obtained from a modality that does not involve radiation. Many pediatric cancer therapy protocols consider CT and MRI to be equivalent in terms of assessing local disease. However, there is little scientific data on which to base a decision regarding the use of CT versus MRI in pediatric abdominal imaging [30]. Although it exposes the patient to radiation, CT offers the ability to cover a large anatomic area, provides excellent spatial resolution with minimal motion artifact and high-quality reconstructed multiplanar images, and is not operator dependant. In contrast, MRI requires substantial technical expertise and long scan times that often necessitate sedation. However, MRI has the important benefit of not utilizing radiation while offering multiplanar imaging with inherent tissue contrast, the ability to characterize tissue with various pulse sequences, and the added potential of providing functional information. The decision to use CT or MRI will depend on the local institution’s standard of practice, the availability of pediatric sedation, and the radiologist’s confidence in performing and interpreting the imaging examination.
Current-day multidetector helical CT (MDCT) scanners allow very rapid image acquisition while maintaining image resolution. These scanners comprise a gantry containing multiple detectors arranged in rows opposite the X-ray tube. The gantry rotates continuously around the patient acquiring data from multiple slices simultaneously as the patient moves through the scanner. Because data are acquired volumetrically, the scan time is shortened while ensuring that small lesions are not missed between slices. Additionally, the quality of multiplanar reconstructed images is greatly improved (Fig. 2.5). Relative to single-slice scanners, MDCT images provide a more accurate assessment of tumor size and a better depiction of the relationship between the tumor and vital structures [5, 31]. Recent advances in MDCT technology include faster gantry rotation times, increased number of detector rows, and dual X-ray tube sources. It is now possible to scan entire body sections in a few seconds or even less than a second. This technology has resulted in a dramatic decrease in the need for sedation while diminishing problems with motion artifact.
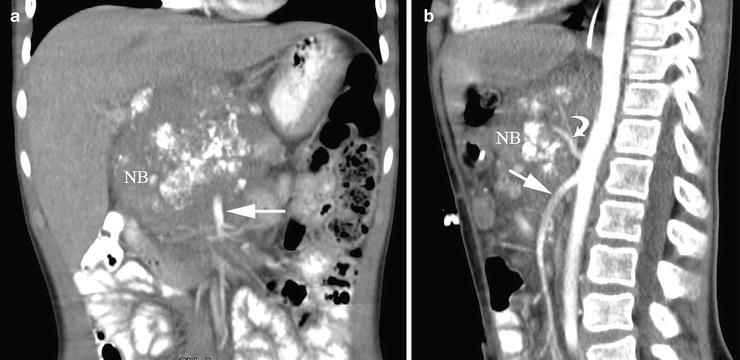
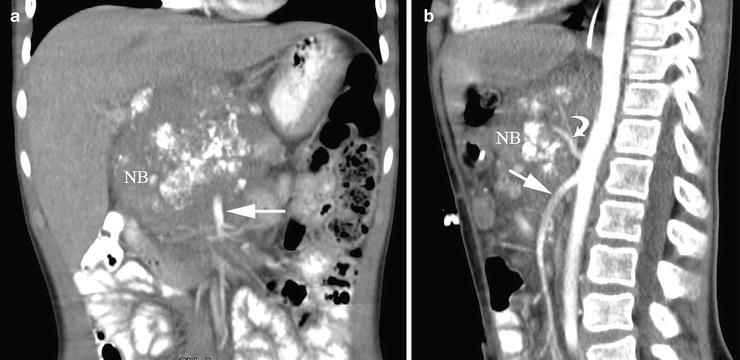
Fig. 2.5
These (a) coronal and (b) sagittal reconstructed computed tomography images provide valuable information regarding the relationship of this neuroblastoma (NB) to the superior mesenteric (straight arrows) and celiac axis (curved arrow) arteries which it encases. Such information is crucial for surgical planning
In the past CT scanning protocols designed for adults were not modified for use in children. With increased awareness and education regarding the harmful effects of radiation from CT scanning in children, significant efforts to minimize radiation dose have been undertaken by industry and the radiology community. Parameters that should be modified for pediatric CT scanning include the tube current (milliampere, mA), tube voltage (kilovoltage peak, kVp), gantry rotation time (second), and pitch.
Tube current has a significant impact on radiation dose and image noise; increases in tube current result in a proportional increase in dose while decreasing current results in increasing image noise. Modern MDCT scanners are equipped with automatic tube current modulation (ATCM) devices that dynamically adjust tube current during scanning in response to the geometry and density of the body part being scanned. The goal of ATCM is to maintain an acceptable image noise level while minimizing radiation dose from tube current [32]. It should be noted that specific pediatric ATCM settings are not universally available on modern scanners and scientific literature regarding appropriate weight, age, body region, and indication-based reference settings is lacking. Therefore, ATCM should be used with care in children [33].
The kVp has an exponential relationship with radiation dose and can substantially reduce radiation dose if optimized. Lowering the tube voltage also lowers the production of scattered radiation. In children weighing ≤45 kg, a tube voltage of 80–100 kVp is usually adequate. For adolescents, a kVp of 100 for the chest and 120 for the abdomen is recommended. Areas with high intrinsic contrast, such as the chest and bones, can be scanned at 80–100 kVp. Recent studies in pediatric phantoms have shown that even lower kVp (approximately 60) may be sufficient for some indications. However, scanner-related parameters, such as tube filtration and scanner geometry, can sometimes negatively impact image quality with lower kVp [33].
Most modern MDCT scanners use rotation times of 0.3–0.5 s resulting in a reduction in radiation exposure, the need for sedation, and motion artifact. Shorter scan times, however, can result in a decrease in the number of profiles that can be used for image reconstruction and, subsequently, an increase in image noise. For optimal image resolution a rotation time of 0.5 s is recommended [32, 33].
The pitch is the ratio between table movement and number of detectors multiplied for section width (collimation). An increase in pitch can result in a reduction in scan time and, in some scanners, a reduction in dose. However, in modern MDCT scanners increasing the pitch can cause a dose increase due to overranging and can also reduce spatial resolution. In general, a pitch of 1–1.5 is currently recommended [32].
Oral contrast material is usually indicated for CT imaging of the abdomen or pelvis and the use of oral contrast material for MDCT is not different than for single-slice CT. Iodinated intravenous (IV) contrast agents should always be used for imaging the neck, abdomen, and pelvis. The use of IV contrast in the chest will depend on the indication for the examination. In our practice, if there is a concern for adenopathy or when there is a primary solid tumor arising in the chest, IV contrast material is used. When chest CT is performed solely to evaluate for pulmonary metastatic disease we do not administer IV contrast material. Due to the very rapid scan times attainable with MDCT scanners it is essential to adjust scanning to allow the IV contrast agent adequate time to reach the area of interest as the area is being scanned. In general, scanning should begin later with MDCT scanners compared to single-slice scanners. Pre-contrast imaging has no role in pediatric oncologic imaging and should not be performed [34]. Post-contrast, multiphase imaging in children is rarely indicated and is also strongly discouraged. An exception is in the evaluation of newly diagnosed liver tumors. The pattern of tumor enhancement on immediate- and delayed-phase post-contrast images can help distinguish hemangiomas from hepatoblastoma. At our institution, we have found that the relationship between a liver tumor and the hepatic and portal veins is best defined when imaging is performed during the arterial and portal venous phases of enhancement. Such information is crucial in determining which Couinaud’s segments are involved and helps guide surgical planning. Specific guidelines for the administration of IV contrast agents and injection techniques (including volume, injection rate, hand injection versus power injector) in children, using MDCT technology, are available in the literature [31].
Magnetic Resonance Imaging
Magnetic resonance imaging plays a pivotal role in the evaluation of newly diagnosed cancer in children. This modality incorporates a strong magnetic field to align hydrogen nuclei within the body. Once aligned the nuclei precess or “wobble” at a frequency proportional to the magnetic field strength. Pulsed radiofrequency (RF) waves are then applied which alter the spin of hydrogen nuclei. When the RF pulse is turned off the nuclei return to their original alignment and energy is released. The released energy is converted to an electrical impulse in a wire within a receiver coil. Spatial encoding is used to localize the site within the body from which the signal originated and, using Fourier transformation (the same mathematical model used to produce a CT image), an MR image is created. Each sampled voxel is assigned a shade of gray that depends on the amount of hydrogen nuclei within it and the rate of equilibrium of hydrogen nuclei back to the original, pre-RF pulse, alignment [5].
Conventional MR imaging relies on several scanning parameters. The RF pulse repetition time (TR) occurs with a time constant, T1. The signal produced in the receiver coil decays exponentially at time constant T2. The time between the initial RF pulse and data collection is the echo time, TE. These parameters can be manipulated so that a T1-weighted (T1W) or T2-weighted (T2W) image is produced. Images acquired with a short TR (300–600 ms) and short TE (10–20 ms) are T1 weighted. On T1W images tissue with short T1 relaxation times (e.g., fat, melanin, gadolinium contrast agent) have high signal intensity and those with longer T1 relaxation times (e.g., water, hemosiderin) have intermediate or low signal intensity. T2W images are produced by using longer TR (>2,000 ms) and a longer TE (>80 ms). On T2W images substances with short T2 relaxation times (e.g., white matter, fibrosis) have low-to-intermediate signal intensity and tissues with longer T2 relaxation times (e.g., edema, tumor, fluid) have higher signal intensity (Fig. 2.6a, b). Additional pulse sequences, beyond these conventional spin-echo sequences, are continually being developed. The inversion recovery sequence (IR) selectively nullifies signal from tissue based on its T1 relaxation time and a selected inversion time (TI). A variant of the IR sequence, the short tau inversion recovery (STIR) sequence, selectively suppresses fat and enhances fluid signal. This sequence has proven valuable in oncologic imaging because tumors, which have high water content, are generally readily visible [5].
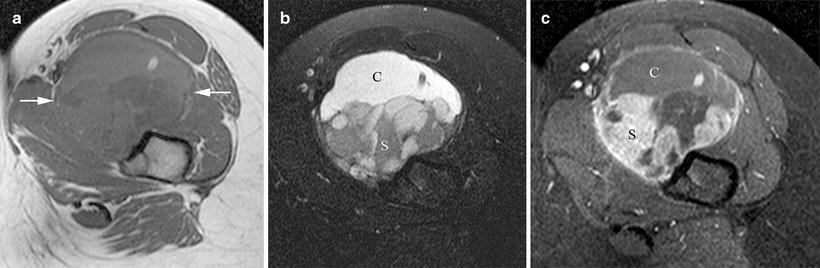
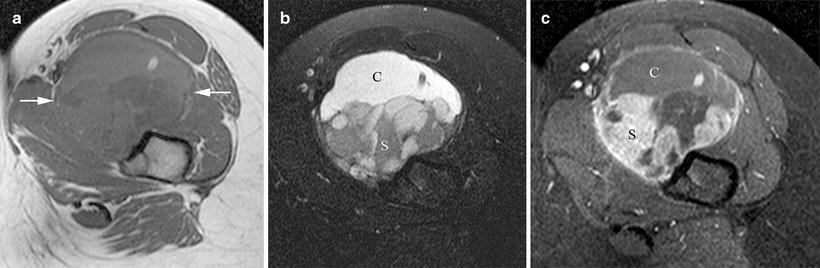
Fig. 2.6
In this patient with a synovial sarcoma the (a) non-contrast-enhanced T1W axial magnetic resonance image shows mixed signal intensity of the tumor (arrows) while the (b) T2W fat-saturated image reveals its partially cystic (C) and solid (S) nature. (c) Post-contrast imaging further delineates the enhancing solid (S) and non-enhancing cystic (C) components
Using various pulse sequences MRI can delineate between normal and abnormal soft tissues better than CT and with the advantage of not exposing the patient to ionizing radiation. In addition to improved soft tissue characterization, with MRI the beam hardening artifact caused by bone on CT imaging is eliminated [5]. These features make MRI the ideal modality for imaging the brain, spine, neck, and extremities. In the past, the multiplanar capabilities of MR, which allow assessment of structures in the axial, coronal, and sagittal planes, were an additional advantage over CT. However, with the advent of MDCT and improved image resolution of coronal and sagittal reconstructed CT images, this advantage no longer holds. A limitation of MR is the long scan times which often require sedation of young patients. Distraction techniques, such as video goggles and audio systems, can help minimize patient motion and avoid the use of sedation in some patients [35]. Long image acquisition times make MRI of lesions in the trunk very susceptible to degradation from respiratory movement, cardiac and vascular pulsations, and bowel peristalsis. Techniques to reduce artifact from bowel peristalsis include keeping the patient from ingesting food or fluid for 4 h before the study and administration of glucagon [35, 36]. Rapid scanning techniques can also help minimize motion artifact. These include low flip angle gradient echo sequences, turbo spin-echo sequences, single-shot sequences, echo planar imaging, and periodically rotated overlapping parallel lines with enhanced reconstruction (PROPELLER) [5, 35]. These faster sequences allow image acquisition during breathholding and coverage of a larger body area in a shorter time period and reduce the potential for motion artifact. Besides the long scan times, other disadvantages of MRI include its high cost, limited availability, relative insensitivity to calcification, and limited ability to assess lung parenchyma [5].
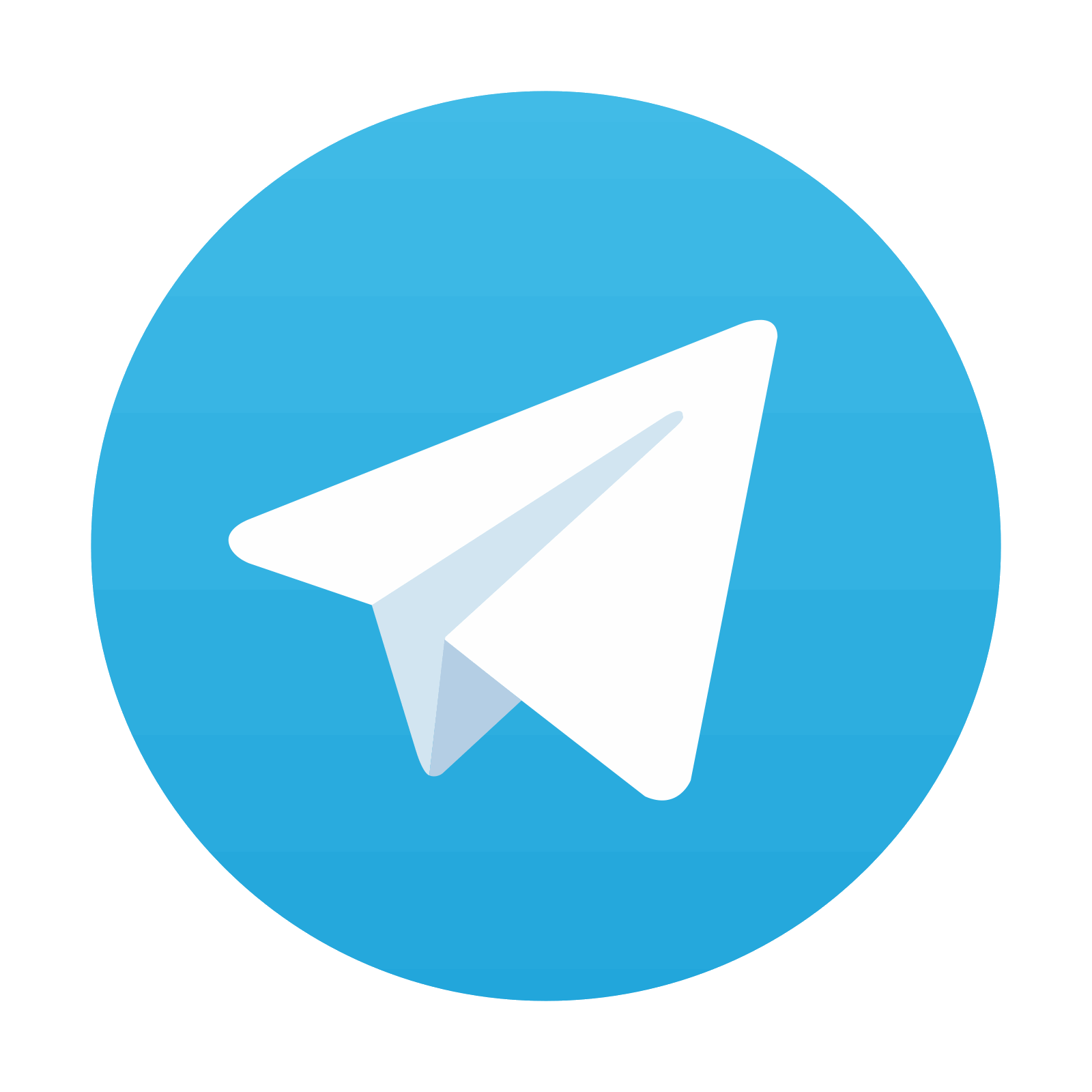
Stay updated, free articles. Join our Telegram channel
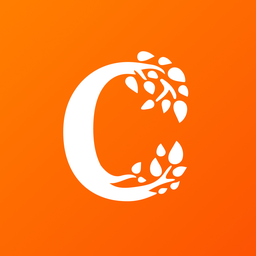
Full access? Get Clinical Tree
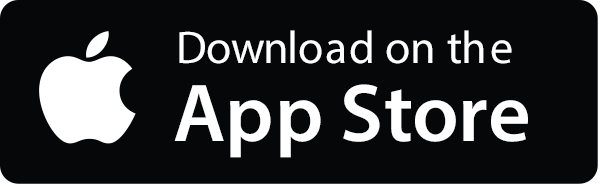
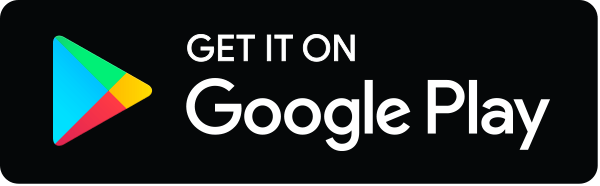