, Abdessalem Rekiki1, Ioanna Theodorou1, Chelsea Samson2, Samantha Blazquez1, Kelly L. Rogers3, Régis Tournebize4 and Spencer Shorte1
(1)
Plate-Forme d’Imagerie Dynamique, Imagopole, Institut Pasteur, Paris, France
(2)
Vanderbilt School of Medicine, Nashville, TN, France
(3)
The Walter and Eliza Hall Institute of Medical Research, Parkville, VIC, Australia
(4)
Plate-Forme d’Imagerie Dynamique, Imagopole, Unité INSERM U786, Institut Pasteur, Paris, France
Abstract
Bioluminescence imaging is a powerful technique that allows for deep-tissue analysis in living, intact organisms. However, in vivo optical imaging is compounded by difficulties due to light scattering and absorption. While light scattering is relatively difficult to overcome and compensate, light absorption by biological tissue is strongly dependent upon wavelength. For example, light absorption by mammalian tissue is highest in the blue-yellow part of the visible energy spectrum. Many natural bioluminescent molecules emit photonic energy in this range, thus in vivo optical detection of these molecules is primarily limited by absorption. This has driven efforts for probe development aimed to enhance photonic emission of red light that is absorbed much less by mammalian tissue using either direct genetic manipulation, and/or resonance energy transfer methods. Here we describe a recently identified alternative approach termed Fluorescence by Unbound Excitation from Luminescence (FUEL), where bioluminescent molecules are able to induce a fluorescent response from fluorescent nanoparticles through an epifluorescence mechanism, thereby significantly increasing both the total number of detectable photons as well as the number of red photons produced.
Key words
FUELBRETQuantum dotBioluminescenceIn vivo1 Introduction
Whole animal bioluminescence imaging (BLI) provides a powerful technological means to observe physiological events inside living systems with minimal perturbation [1–5]. It therefore has utility in studies on, for example, tumor development [3, 6], immunity [4], infection [7], gene expression [8], and inflammation [9]. However, a critical challenge for in vivo BLI comes from an abundance of endogenous optical absorbers, such as hemoglobin [2, 10, 11]. Hemoglobin readily absorbs blue-yellow light (400–650 nm); strongly overlapping the most commonly used bioluminescent sources (i.e., Photorhabdus luminescens, Gaussia, and even firefly luciferase to some extent). Consequently, the light output of luminescent probes can be quenched, and/or scattered by tissues, eventually resulting in a reduced amount of detectable signal. Likewise, these same constraints are encountered under in vitro conditions using animal extracts such as blood or homogenized tissue. Because biological tissue is more permissive to red light, much effort has been directed towards improving BLI sensitivity by red-shifting the emission wavelengths to the desired optical window of 650–900 nm, a range in which optical absorption is minimized significantly [12]. To achieve this, researchers have tried either to create mutations of the common luciferases (for example, the work by Branchini et al.) [12], or by proxy using bioluminescence resonance energy transfer (BRET) [13–15].
Unlike classical fluorescence excitation, where excitation light radiatively excites a fluorophore, BRET is a process by which the photon energy of a luminescent “donor” molecule can pass directly (via a non-radiative resonance energy transfer, RET) to a fluorescent “acceptor” molecule within 10 nm proximity, resulting in fluorescence. The Stokes shift of the fluorophore yields fluorescence emission at a red-shifted, longer wavelength than the original luminescence. Recently we reported an alternative method to achieve a similar red shifted emission by using Fluorescence by Unbound Excitation from Luminescence (FUEL) [2, 10]. The FUEL method differs from BRET because it arises from an irradiative epifluorescent excitation/emission interaction between the bioluminescent molecule and fluorophore. The experimental conditions favoring FUEL are very similar to those for BRET: a strong spectral overlap between the bioluminescent source and the fluorophore must exist. The major difference is the distance tolerance over which the phenomena may occur. The working distance of FUEL is much greater than 10 nm. Under in vitro conditions and in the absence of an optical absorber, the FUEL effect has been observed with up to 3 cm between the luminescent source and the fluorophore moieties, without covalent coupling chemistries or surface modifications of either of the FUEL components.
The differences between FUEL and BRET underline their distinct utility as experimental tools. While the use of BRET is well established in the literature, we propose that FUEL brings a different utility to the experimental toolbox for both in vitro and in vivo applications. For example, the possibility to measure entity co-proximity at a macroscopic level would be invaluable. Towards these ends, we present in detail simple and robust methods to reconstitute FUEL using bioluminescent Escherichia coli and quantum dots, under controlled in vitro and in vivo demonstrations. With the principles demonstrated here it should be possible to extrapolate the appropriate techniques for more complicated in vitro or in vivo applications thereof.
2 Materials
1.
Standard bacteria culture media (LB for example).
2.
Bioluminescent E. coli carrying the plasmid expressing the lux operon (DH5alpha carrying pUC18-mini-Tn7T-Gm-lux; referred heron as RT57) with an OD600 = 2 (for the RT57 E. coli, an OD600 = 1 is 5 × 108 bacteria per ml, see Note 1). Other luminescent bacterial sources may be used such as the commercially available Staphylococcus aureus Xen 36 (PerkinElmer, USA).
3.
Physiological saline (0.09 % NaCl, PS); may be prepared in-house or purchased.
4.
Qtracker 705 (2 μM, QD705) non-targeted quantum dots (Invitrogen). Keep in the dark at 4 °C until use. The QD705 are used as received.
5.
A black-walled 96-well plate.
7.
Agarose (Sigma), prepared into 3 % and 4 % solutions.
8.
For all animal experiments, female 6-week-old BALB/c mice ( Janvier, France) are used.
9.
An electric razor equipped with a fine blade.
10.
Commercial epilation cream such as Nair®.
11.
Standard chemical anesthesia solution, used at 1.5 mg/kg Ketamine and 30 mg/kg Xylazine.
12.
All animal experiments should be carried out only under strict accordance with applicable national and institutional guidelines and only by suitably accredited, qualified personnel.
2.1 Bioluminescence Imaging
1.
An IVIS 100 whole animal imaging system (Xenogen Corporation, Caliper Life Sciences, a PerkinElmer Company, Alameda, CA) equipped with an intensified deep-cooled CCD and a filter wheel is used to acquire all bioluminescent and fluorescent images.
2.
One location within the filter wheel is kept empty for total light detection (Total Light).
3.
A 480 nm ± 10 nm band pass filter is used to collect the RT57-specific photons.
4.
A Cy5.5 band pass filter (695–770 nm) is used to distinguish red photons.
5.
The CCD is cooled to −105 °C and the stage warmed to 37 °C for each experiment.
6.
For each image, the acquisition settings for the Living Image (Xenogen) software version 3.1 were set as follows: 8× bin, field of view B (20 cm), and f-stop 1.
7.
The acquisition time is typically 10–60 s, but can be adjusted as necessary.
8.
The illumination power was set to low, the bin setting was reduced to 4×, and the acquisition time set to 0.5 s for fluorescence image acquisitions.
9.
QD705 fluorescence was observed using a Cy5.5 filter set that included a 615–665 nm excitation filter and a 695–770 nm emission filter (see Note 3).
3 Methods
3.1 Demonstration of FUEL in Suspension
This initial experiment attempts to demonstrate the basic building blocks of the FUEL phenomenon. The existence of resonance energy transfer between two unbound moieties is minimal. The event becomes even more unlikely when bacteria are the luminescent source. This is due to the fact that the luminescence evolves from below the inner membrane of the bacteria and that the fluorophore, QD705 in this case, remains exterior to the bacteria obscuring any possibility of binding between the two components occurs. Given the need for the luminescent/fluorescent molecules to be within 10 nm proximity for RET to occur, one can conclude that any detected red signal is due exclusively to FUEL.
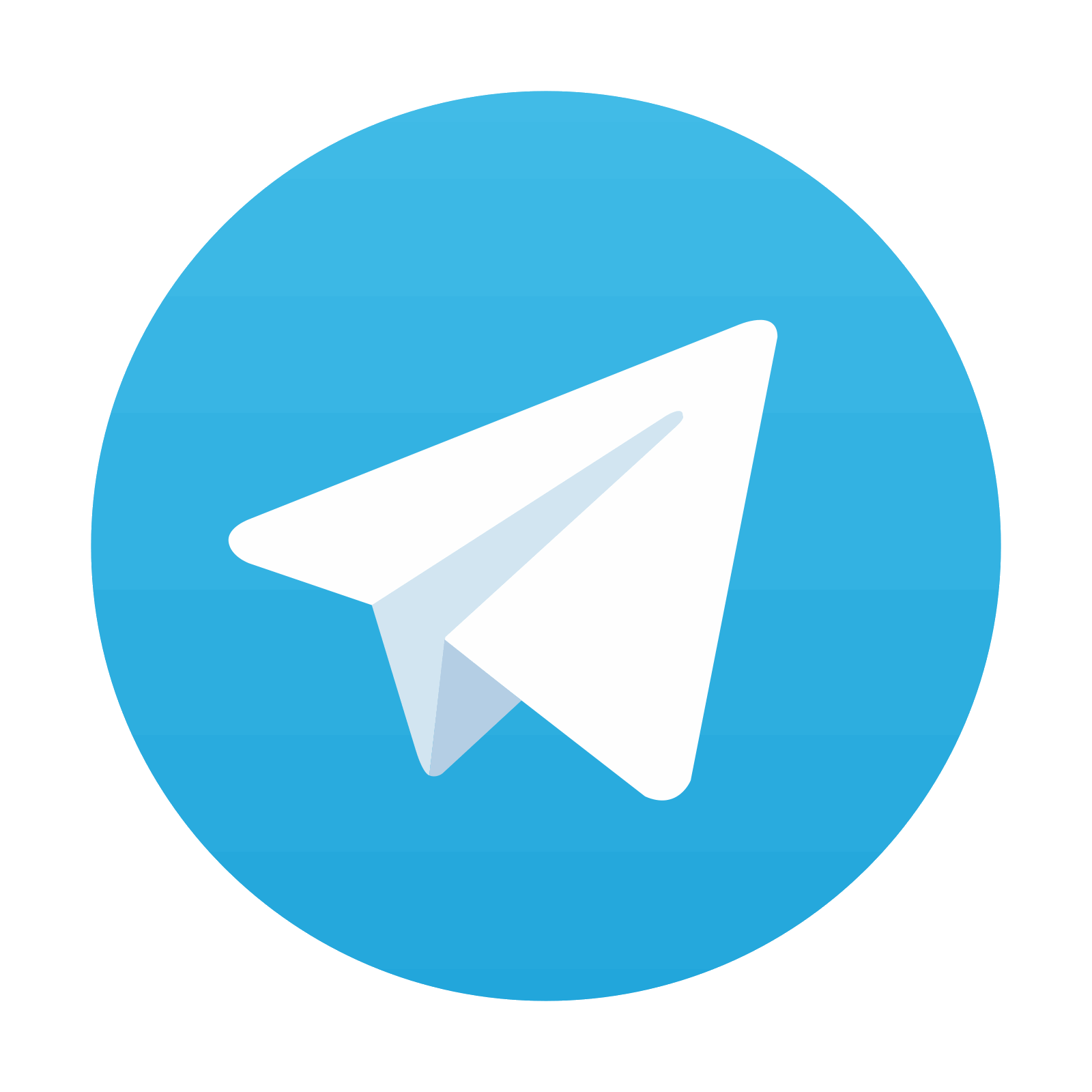
1.
Prepare an overnight culture of the appropriate bacteria at the appropriate temperature, typically reaching an OD = 2–3 by the next morning. Then, seed subcultures as needed in fresh media until OD ~ 1/mid log phase is reached. Finally, dilute or concentrate if necessary to reach a final concentration of 5 × 108 bacteria/ ml
2.
Label three tubes: “Bac,” “QD705,” “Bac + QD705”
3.
Add 90 μl of PBS or physiological saline (PS) and 10 μl of the bacterial stock to the Bac tube. Then, into the QD705 tube, add 95 μl of PS and 5 μl of QD705. Finally, add 85 μl of PS, 10 μl of the bacteria stock solution, and 5 μl of QD705 to the Bac + QD705 tube.
4.
Distribute the prepared tubes into individual wells of a black-walled 96-well plate (Fig. 1).
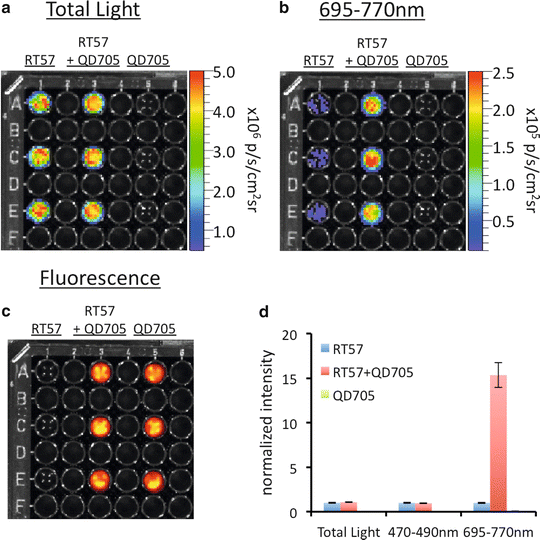
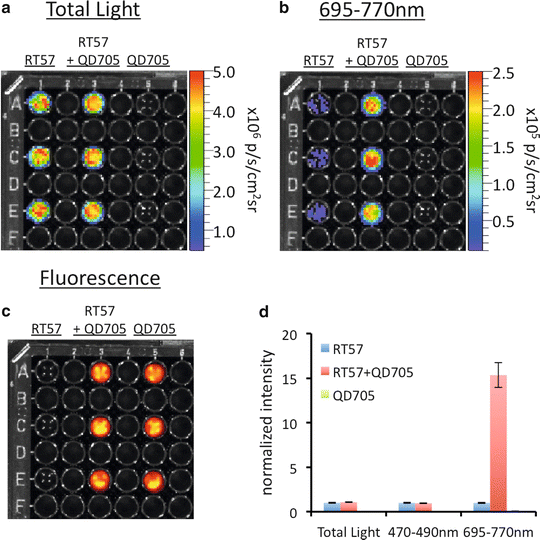
Fig. 1
A demonstration of FUEL occurring between luminescent bacteria and nanoparticles in suspension. Solutions of bacteria (Bac), bacteria with QD705 (Bac + QD705), and QD705 alone (QD705) were prepared in triplicate as described in the text and then distributed individually into a black-walled 96-well plate. The plate was then observed using an IVIS100 under the (a) total light, 470–490 nm band pass filter (not shown), and the (b) 695–770 nm band pass filter. The location of the QD705 was confirmed by epifluorescent excitation (c). Each individual well from (a) and (b) were normalized to the average of the Bac control under each respective filter set, with the relative intensity and standard deviation represented in (d), n = 3
5.
Repeat steps 2 and 3 for as many replicates as desired using a unique bacterial culture each time.
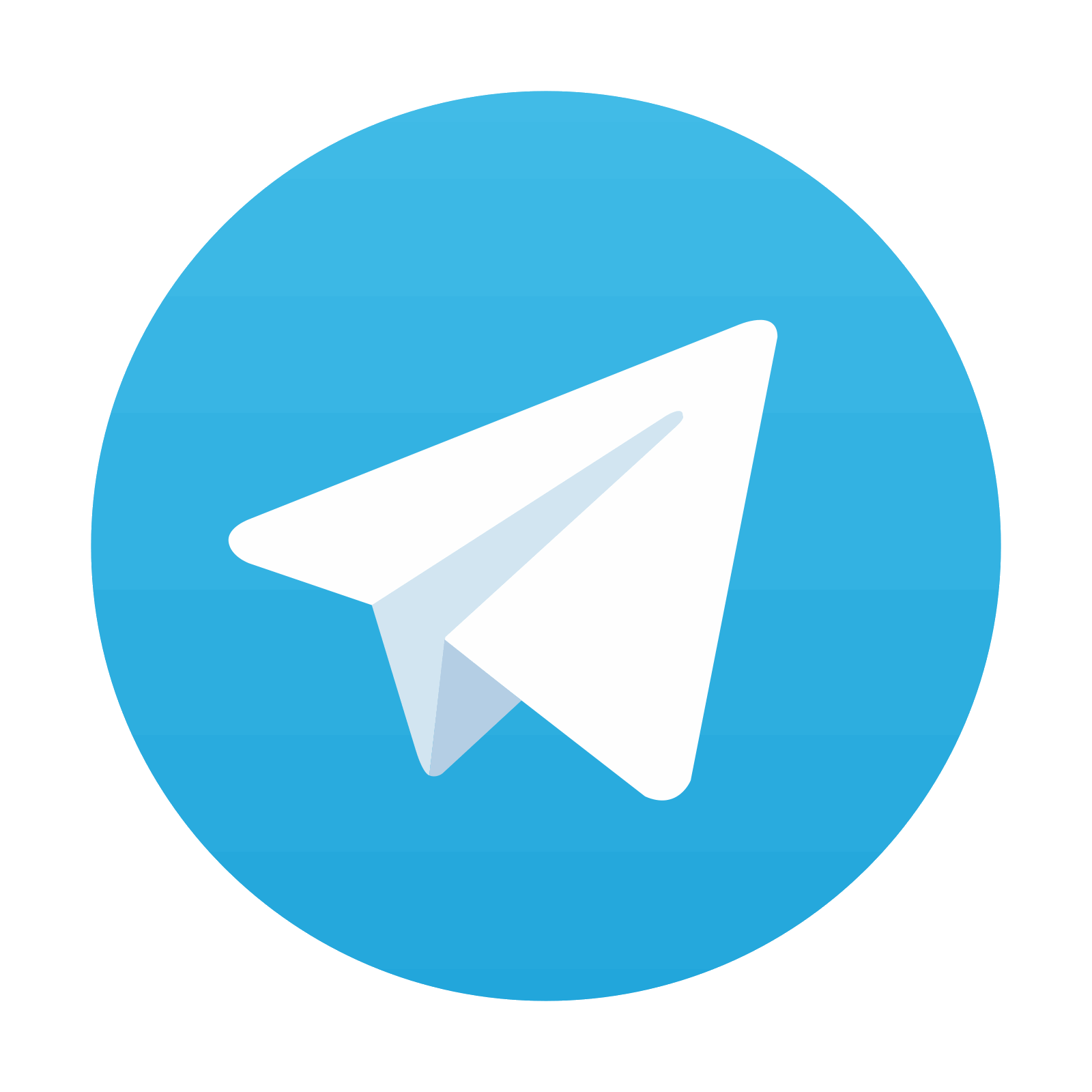
Stay updated, free articles. Join our Telegram channel
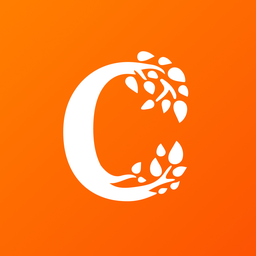
Full access? Get Clinical Tree
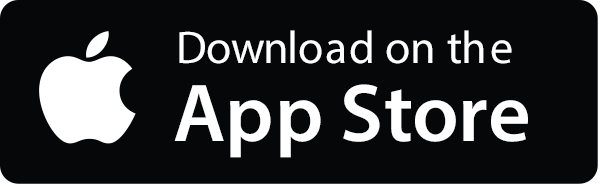
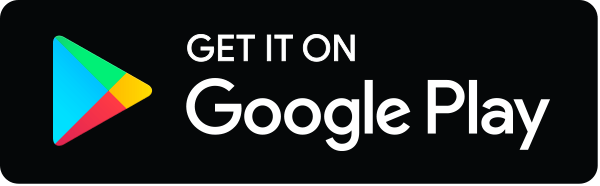
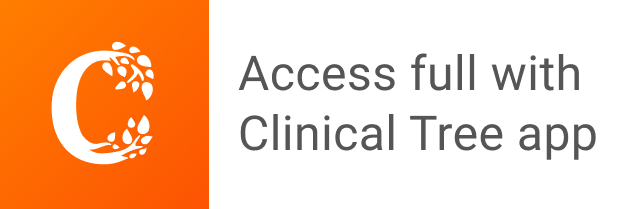