Fig. 10.1
A schematic depiction of the interaction of nanoparticles with ionizing radiation [24]. With permission from J.W. Bergs et al.
Safety and Potential Toxicity of Nanoparticles
For the successful clinical translation of nanoparticles, as with any medicine, thorough and careful evaluation of both the safety and pharmacokinetics of the agent is needed. Analysis of nanomaterial toxicity can be done using either in vitro or in vivo methods. The in vitro approach is by far the most commonly used as results can be determined rapidly at a low cost without the use of animals and can provide some insight into the biocompatibility of a nanoplatform. Some commonly accepted methods include the MTT assay for mitochondrial function , the clonogenic assays for cell proliferation and colony studies, and the lactate dehydrogenase assay for evaluating the integrity of the cell membrane, as well as using immunohistochemistry markers for measuring apoptosis and necrosis. While these methods are effective for providing some guidance of potential toxicity profiles, the in vivo interaction of nanoparticles with complex and dynamic biological systems cannot be predicted with substantial accuracy. Therefore, in vivo testing of nanoparticles is often done to determine the pharmacokinetic and pharmacodynamic profile and to understand their biocompatibility and safety. Methods for in vivo evaluation include determining organ biodistribution using multiple time points, blood sample collections for the analysis of circulation half-lives and liver enzymes, changes in appetite or weight, inflammatory cytokines, and histological tissue sectioning for microscopic examination to organ-specific toxicity . Additionally, blood chemistry analytes exist for the evaluation of specific organ toxicity such alanine aminotransferase (ALT), aspartate aminotransferase (AST), alkaline phosphatase, and total bilirubin for the evaluation of the hepatobiliary system and potential hemolysis.
When designing a nanomedicine for clinical translation, careful consideration of the factors and components that are responsible for the generation of toxicity are in order to maximize the chances of creating a safe agent. For example, silver is generally considered nontoxic when used on a large scale but can be toxic when used on a nanoscale [28]. Furthermore, the pharmacokinetic and pharmacodynamic profiles may provide a basis for potential fates and effects within the human body. For example, particles that are removed by the RES have the potential to cause toxicity and damage in those tissues involved in the clearance of the nanoparticles (liver, spleen, bone marrow). Therefore, the safety of nanoparticles will depend on many parameters including the chemical composition of the nanoformulation, size, shape, reactivity, stability, surface coating, and charge. One must therefore take into account all these properties when evaluating the safety and biocompatibility of nanoparticles.
The general rule of thumb for limiting the potential for nanoparticle toxicity as it relates to nanoparticle size is that they are inversely proportional to one another. This is because nanoparticles become more reactive as they become smaller, and their surface area to volume ratio increases. In addition to size, the nanoparticle shape and surface charge can also contribute to nanoparticle-induced toxicity. Studies have shown that the shape of nanoformulations dictates resulting interactions with biological systems including diffusion, translocation across cell membranes, and biodistribution [29]. For instance, a study evaluating cellular uptake of nanoparticles has shown that spherical AuNPs have a higher uptake in cells compared to gold nanorods [30]. With respect to surface charge, particles with a net negative surface charge tended to be less toxic than those with a positive surface charge, since cell membranes are negatively charged and positively charged particles are taken up by cells more readily. This concept can be exploited to help improve nanoparticle transportation into cancer cells. In one example, nanoparticle surfaces can be linked with a neutral compound that can become positively charged within low-pH microenvironments of certain tumors enabling local intracellular delivery of payload [31]. Surface coating is another important characteristic to consider since it can affect nanoparticle surface charge, hydrophilicity, hydrophobicity, protein adsorption, circulation half-lives, and interaction with specific cell types [32]. The final aspect to take into consideration is nanoparticle stability. This is relevant since nanoparticles can break down in the harsh, acidic environment of lysosomes increasing the concentration of toxic ions within cells, resulting in the buildup of reactive oxygen species [33]. The main mechanisms through which nanoparticles have the potential to exert a toxic effect on biological structures include the generation of free radicals and reactive oxygen species [34], or altering the binding stability and catalytic activity of protein structures, which can ultimately result in the induction of inflammation, genotoxicity, cytotoxicity, and developmental abnormalities [35].
While these nanoparticle characteristics are useful for predicting the potential for toxicity, a clear-cut correlation may not always exist across different nanoparticle platforms and other materials. For example, iron nanoparticles are generally regarded as safe and have been approved by the Food and Drug Administration for the treatment of anemia and contrast-enhanced MRI imaging [36, 37]. On the other hand, it was found that inclusion of safe iron oxides in emulsions made from edible oils resulted in nanoparticles that could produce toxicity, since the iron oxides catalyzed the oxidation of the oils to produce toxic substances [38]. Similarly, gadolinium used clinically as an MRI contrast agent is well tolerated; however, in patients with compromised kidney function gadolinium, exposure can result in nephrogenic systemic fibrosis [39]. Gold is considered to be very safe. In fact, gold has been used in medical practice throughout history and continues today as a treatment for rheumatoid arthritis [23]. Accordingly, when 12.5 nm AuNPs were administered intraperitoneally into mice every day for 8 days, no evidence of toxicity was observed in any of the studies performed, including survival, behavior, animal weight, organ morphology, blood biochemistry, and tissue histology [40]. In addition studies utilizing 1.9 nm and 0.8 nm AuNPs did not suggest any toxicity in mice [41]. In another study, a toxicological analysis of mice evaluating the intravenous injection of 0.9 nm and 5 nm up to 3 months showed no signs of illness and revealed blood chemistry values within normal limits [42]. Numerous other studies also support the assertion that AuNPs are not toxic to cells [43–48].
Nanoparticles in Radiation Therapy
Since current irradiation strategies may fail to kill all cancer cells within an irradiated volume, it may be beneficial to selectively enhance radiation at the cellular level. Consequently, many approaches have been developed to enhance the radiation effects specifically within tumors. A radiosensitizer is an agent or drug that increases the cytotoxic susceptibility of cancer cells to radiation therapy. Ideally a radiosensitizer would act specifically on tumor cells while sparing normal tissues, have favorable pharmacokinetic profiles for tumor accumulation prior or during radiation therapy, and be nontoxic. A variety of approaches have been implemented to increase radiation response to help decrease cellular resistance to ionizing radiation while minimizing toxicity to normal tissues. These include oxygen imitators [49–51], thymine analogues [52], inhibitors of cellular repair and cellular processes [53–56], thiol scavengers [52], and nanoparticles [25]. Among these, nanoparticles are favorable because they are able to increase tumor penetration, reduce required radiation doses thereby minimizing adverse effects compared to conventional radiosensitizers , and have been shown to be a promising strategy for increasing the efficiency of radiation therapy [57]. Studies have shown that nanoparticle carriers formed from poly(lactic-co-glycolic acid) PLGA , a biodegradable polymer that can be easily hydrolyzed into the metabolites lactic acid and glycolic acid, containing paclitaxel and etanidazole are able increase radiation sensitivity in tumor cell lines compared to free drug alone or nanoparticles containing only one of the agents [58]. Furthermore, nanoparticles have been used to encapsulate the poorly water-soluble radiosensitizer docetaxel to circumvent the undesirable side effects associated with administration of free drug [59]. Another polymeric nanoparticle that has shown to be a more effective radiation sensitizer in vivo compared to free drug alone is Genexol-PM , a polymeric micelle containing paclitaxel used for the treatment of non-small cell lung cancer [60]. However, the most extensively studied nanoparticles for radiation enhancement are those with high Z numbers. For example, gold [61], gadolinium [62], bismuth [63], titanium [64], hafnium [65], germanium [66], and platinum [67] have been evaluated for their radiosensitization capabilities. This is because high Z materials have a higher probability of emitting auger electrons and photoelectrons producing highly oxidizing free radical molecules that cause cellular death. Of all the high Z material nanoparticles , AuNPs have been the most thoroughly evaluated. The next section will focus primarily on radiation therapy involving nanoformulations containing AuNP.
Mechanisms of Interaction of Radiation with Nanoparticles
The primary objective of radiation therapy is to deprive cancer cells of their mitotic potential and ultimately promote cancer cell death. The main interaction of X-rays in cells is by Compton scattering , producing secondary high-energy electrons that exert their effects on biological structures. In the cell, DNA is the desired biological target of ionizing radiation. There are two mechanisms by which radiation can interact with DNA. The first is known as direct action where ionizing radiation interacts directly with DNA to cause damage. The second is known as indirect action where ionizing radiation interacts with the surrounding water molecules, generating free radicals, notably hydroxyl radicals [68], which cause lethal damage to cellular DNA. Hydroxyl radicals are generated either directly by the oxidation of water by ionizing radiation or indirectly by the formation of secondary partially reactive oxygen species (ROS) . ROS include superoxide (O2−), hydrogen peroxide (H2O2), and hydroxyl radicals (OH═). The damage caused can include DNA strand breaks that are initiated by the removal of a deoxyribose hydrogen atom by the activated hydroxyl radical [69]. Excessive damage to cells exposed to radiation can lead to either double-strand breaks (DSB) or single-strand breaks (SSB) . DSBs are the not the most common type of radiation-induced damage but are regarded as the most serious and potentially lethal. At this stage, some cells will arrest their cell cycle to repair the damage. If the damage is beyond repair then the cell will undergo apoptosis. Alternatively, some cancer cells with mutations in cell cycle checkpoints can continue to proliferate following radiation exposure. However, the majority of these cells will undergo cell death during mitosis as a result of sustained DNA damage and chromosomal defects. The postmitotic or reproductive mode of cell death is considered to be the most prevalent mechanism in cells exposed to ionizing radiation [70–72]. The apoptotic signaling pathway can be initiated in various cellular compartments that include the plasma membrane, cytoplasm, and nucleus [73]. In the plasma membrane, ionizing radiation can promote lipid-oxidative damage through interactions with radiation-induced free radicals resulting in altered ion channels, a buildup in arachidonic acid, and the production of ceramide which is involved in mediating cellular death. Cell death occurs via free radical molecules eliciting cumulative un-repairable lipid-oxidative damage [75].
The mechanism of nanoparticle enhancement, in X-ray therapy, is dependent on the energy of incident ionizing photons and different interactions between the photons and nanoparticles. The three fundamental mechanisms of radiation enhancement are the photoelectric effect , Compton scattering , and pair production . The photoelectric effect is the predominant mechanism of radiosensitization of high atomic number (Z) elements, for photons with energies in the range of 10–500 keV [76]. The cross section of the photoelectric effect varies with the atomic number approximately proportional to Z3, meaning that higher Z atoms will have a larger absorption cross section. The photoelectric effect is also dependent on the energy of the photon, with a maximum cross section when the photon energy is equal to the binding energy of orbital electrons. This effect decreases sharply as energy is increased and varies as E−3. For example, the binding energies of electrons bound to gold are 79 keV for the inner shells, 13 keV, and 3 keV for outer shells, while those of soft tissue are on the order of 1 keV or lower resulting from the lower atomic number of organic matter. Therefore, gold would absorb significantly more energy than soft tissue in the kilovoltage energy range. When photons with energies in these ranges interact with AuNPs, they can produce electrons, characteristic X-rays of gold atoms , or Auger electrons . Once an atom absorbs a photon, an electron may be emitted resulting in an ionized atom.
When photons of energy greater than the binding energy of an inner shell electron collide , that electron is ejected leaving behind a vacancy in an orbital electron shell. As a result, outer electrons in a higher-energy state fill the vacancy in the lower-energy orbital. This process is accompanied by either a fluorescent photon or an Auger electron ejected from an outer shell with an energy equal to the difference between the two orbital shells. If multiple shells exist within an atom, then further Auger electrons can be generated as outer shell electrons fill in the vacancies. This phenomenon is known as the Auger cascade . The number of Auger electrons emitted is directly proportional to the atomic number. Therefore, high Z atoms are expected to generate more Auger electrons than elements with lower atomic numbers [77]. The range of these emitted electrons has been calculated to be around tens of nanometers depositing their energy along their path and distributing radiation throughout the system [77]. Furthermore, the Auger electron “shower” can produce highly positively charged ions, causing local Coulomb force fields that can disrupt nearby cellular structures.
The enhancement of radiation with high Z material was first realized when DNA damage was detected in lymphocytes isolated from patients receiving iodinated contrast agents for X-ray imaging [78]. Since then many other studies have demonstrated that radiation therapy in combination with iodine suppresses tumor growth and improves survival in animal models [79]. Another interesting approach was the incorporation of iodine into cellular DNA yielding a threefold improvement in in vitro radiosensitization [80]. However, this strategy is not as effective if insufficient levels of thymine are substituted with iododeoxyuridine. Although the mechanisms of radiation enhancement of gold nanoparticles are not completely understood, it is currently believed that the interaction of X-rays with high Z atoms induces the release of photoelectrons and Auger electrons [76] (Fig. 10.2).
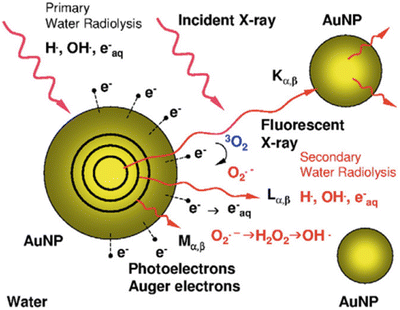
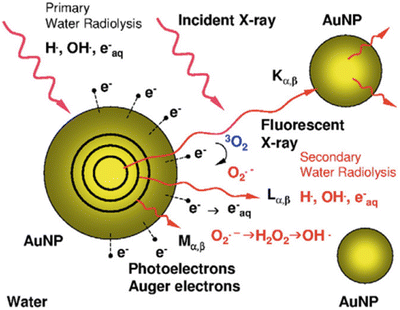
Fig. 10.2
Schematic depiction of increased generation of reactive oxygen species by the emission of photoelectrons and Auger electrons from AuNPs in the presence of ionizing radiation [74]
Given that gold has a higher Z number (79 vs 53), it is likely that gold as a radiosensitizer would be much more effective than iodine. When photon energies are greater than 500 keV, Compton effects begin to dominate. The Compton effect is the incoherent or inelastic scattering between an X-ray photon and an electron of an atom. In this interaction, only a part of the energy is transferred to the electron. The resulting emitted electron is known as a Compton electron , leaving behind an ionized atom or molecule. In contrast to photoelectric interactions where most photoelectrons are inner electrons, Compton interactions increase for loosely bound electrons. So most of the Compton electrons are valence electrons. In contrast to Auger electrons , Compton electrons are capable of traveling several hundred microns. For incident photons with energies higher than 1.02 MeV, a process known as pair production dominates where the photon is absorbed by the nucleus with the production of a positron and electron pairs. The probability of pair production increases with the atomic number as Z2 and linearly with the energy of incident photons. The interaction of charged particles is more complex; however, some studies have speculated that proton-AuNP interactions lead to the increased production of low-energy delta-ray electrons producing a high degree of lethal damage within the cells, thus lowering the surviving fraction of cells [81].
While most nanoparticle radiosensitization has primarily been attributed to their photon absorption capabilities, recent studies highlight that a significant biological component may be responsible for radiosensitization . In the absence of radiation, nanoparticles have been reported to induce ROS that cause oxidative DNA damage [82]. In addition, nanomaterials have been shown to cause alterations in the cell cycle with an increase in cells at the G2/M phase [83]. In a recent study by Kang et al., the nuclear targeting of AuNPs was shown to cause cytokinesis arrest leading to the failure of complete cell division and apoptosis [84]. Although experimental evidence may suggest the involvement of biological components in radiosensitization , the exact mechanisms are still not clearly understood.
In Vitro Radiosensitization Using AuNPs
By far the majority of in vitro and in vivo studies analyzing AuNP-mediated radioenhancement rely on passively targeted nanoparticles. One of the earliest studies using gold for radioenhancement was performed by Regulla and colleagues [85]. In this study, enhanced radiation effects were observed in mouse embryo fibroblasts that were exposed to gold surfaces compared to those exposed to polymethyl methacrylate . Secondary electrons were found to travel a range of approximately 10 μm. Following this study, numerous other experimental studies using AuNPs over both orthovoltage (200–500 keV) and megavoltage (>100 keV) ranges have been described. The results of these reports are difficult to compare directly since they were performed using many parameters such as size, shape, surface coating, concentration, radiation type and energy, and origin of cell lines (Table 10.1 adapted from Butterworth et al.). In an attempt to address these issues, Brun and coworkers investigated AuNP radiation enhancement by systematically altering AuNP concentrations, AuNP diameter, and incident X-ray energy (range 14.8–70 keV). They determined that the conditions with the most radiation enhancement were those using larger sized AuNPs , high gold concentration, and 50 keV photons providing dose enhancement factors of 6 [98]. In a separate study, 1.9 nm AuNPs enhanced the response of bovine aortic endothelial cell damage inflicted by X-ray irradiation, with a dose enhancement factor up to 24.6 [91]. While optimal sizes for AuNP radiation therapy may be inconclusive, it is generally accepted that radiation-induced DNA damage will increase with increasing concentrations of AuNPs [99]. In vitro experiments using brachytherapy sources and AuNPs have also been reported and initially demonstrated an increased biological effect with irradiation with values up to 130 % greater than without AuNPs [100].
Table 10.1
Summary of in vitro radiosensitization experiments using AuNPs
Author | Size (nm) | Concentration | Surface coating | Cell model | Energy source | DEF | SER |
---|---|---|---|---|---|---|---|
Geng et al. [86] | 14 | 5 nM | Glucose | SK-OV-3 | 90 kVp | 1.002 | 1.3 |
6 MV | 1.00009 | 1.2 | |||||
Jain et al. [87] | 1.9 | 12 μM | Thiol | DU-145 | 160 kVp | 1.05 | <1.41 |
MDA-231 MB | 6 MV | 1.0005 | <1.29 | ||||
L132 | 15 MV | 1.0005 | 1.16 | ||||
6 MeV e− | 1 | <1.12 | |||||
16 MeV e− | 1 | 1.35 | |||||
Chithrani et al. [79] | 14 | 1 nM | Citrate | HeLa | 220 kVp | 1.09 | 1.17–1.16 |
74 | 6 MV e− | 1.0008 | |||||
50 | 662 keV | 1.0006 | |||||
Liu et al. [88] | 6.1 | >1 mM | PEG | CT-26 | 6 keV e− | 1 | 2 |
EMT-6 | 160 kVp | 1.02 | 1.1 | ||||
6 MV | 1.002 | 1 | |||||
Butterworth et al. [89] | 1.9 | 2.4 μM | Thiol | DU-145 | 160 kVp | 1.01 | <1 |
MDA-231 MB | |||||||
0.24 μM | |||||||
AG0-1522 | |||||||
Astro | |||||||
L132 | 1.01 | <1.67 | |||||
T98G | 1.01 | <1.97 | |||||
MCF-7 | |||||||
1.01 | <1.04 | ||||||
PC-3 | 1.01 | <1 | |||||
1.01 | <1.91 | ||||||
1.01 | <1.41 | ||||||
1.01 | <1.07 | ||||||
1.01 | 1.3 | ||||||
Kong et al. [90] | 10.8 | 15 nM | Glucose | MCF-7 | 200 kVp | 1.01 | 1.3 |
662 keV | 1.00008 | 1.6 | |||||
Cysteamine | MCF-10A | 1.2 MV | 1.00001 | ||||
Rahman et al. [91] | 1.9 | <1 mM | Thiol | BAEC | 80 kV | 6.6 | 20 |
150 kV | 5.2 | 1.4 | |||||
6 MV e− | 1 | 2.9 | |||||
12 MV e− | 1 | 3.7 | |||||
Roa et al. [83] | 10.8 | 15 nM | Glucose | DU-145 | 662 keV | 1.00008 | >1.5 |
Zhang et al. [92] | 30 | 15 nM | Glucose-TGS | DU-145 | 200 kVp | 1.0083 | >1.3 |
1.0083 | >1.5 | ||||||
Chang et al. [93] | 13 | 11 nM | Citrate | B16F10 | 6 MV e− | 1 | 1 |
Chien et al. [94] | 20 | <2 mM | Citrate | CT-26 | 6 MV e− | 1 | 1.19 |
Zhang et al. [95] | 4.8 | 0.095–3 mM | Citrate | K562 | 2–10 kR gamma | ||
12.1 | |||||||
27.3 | |||||||
46.6 | |||||||
Liu et al. [96] | 4.7 | 500 μM | PEG | CT-26 | 6 MV | 1.3–1.6 | |
Chattopadhyay et al. [97] | 30 | 0.3 nM | Trastuzumab-PEG | SK-BR-3 | 300 kVp | 5.1 | |
Brun et al. [98] | 8.1 | 1–5 nM | Citrate | Plasmid DNA | 30 kV | <3.3 | |
20.2 | 80 kV | ||||||
37.5 | 80 kV | ||||||
74 | 100 kV | ||||||
91.7 | 120 kV | ||||||
150 kV |
Most photoelectrons , Auger electrons , and other secondary electrons have low energies and a short range in tissues (nm to μm) delivering lethal doses in their immediate surroundings [101]. The possibility of having AuNPs target specific cancer cells may increase the production of secondary electrons within the vicinity of DNA molecules, especially if they involve cellular internalization [102]. Chattopadhyay et al. was one of the first to validate this hypothesis by synthesizing trastuzumab-PEG-AuNPs [97]. Briefly, SK-BR-3 cells were irradiated after treatment with either phosphate-buffered saline, PEG-AuNPs, or trastuzumab-PEG-AuNPs. The DNA DSBs as measured by γ-H2AX foci increased 5.1 and 3.3 times for targeted AuNPs compared to cells treated with PBS or PEG-AuNPs, respectively. AuNPs modified with either cysteamine or thioglucose have been shown to have differential accumulation in cancer cells. While cysteamine-modified AuNPs were preferentially limited to the cell membrane of MCF-7 breast cancer cells, glucose-AuNPs are internalized and distributed throughout the cytoplasm [86, 90]. Furthermore, glucose-AuNPs exhibited enhanced irradiation (200 kVp)-induced cell death compared to cysteamine-AuNPs and irradiation alone. Finally, in an independent study, the radiotoxicity of proton therapy with AuNP internalization was increased by approximately 15–20 % compared to proton therapy without AuNPs [81]. However, the meaning of these results is not clear, as targeted AuNPs were not compared to nontargeted AuNPs.
In Vivo Radiosensitization Using AuNPs
In 2004, Hainfeld et al. performed the first animal study evaluating enhanced tumor radiosensitization via AuNPs . Using 1.9 nm AuNPs in combination with 250 kVp X-rays (30 Gy), overall tumor-xenograft mouse survival was 86 % versus 20 % for radiation alone and 0 % for gold only [103]. Since then AuNP radiosensitization has been demonstrated in vivo with murine mammary ductal carcinoma [104], murine squamous cell carcinomas [103], human sarcoma cells [105], and cervical carcinoma (see Table 10.2) [111]. In a study by Zhang and colleagues, in vivo radiosensitization was studied using four different sizes of PEG-AuNPs, and demonstrated that while all sizes can decrease tumor volumes after gamma radiation (5 Gy), the smallest (4.8 nm) and largest (46.6 nm) particles tested had weaker sensitization effects than 12.1 and 27.3 nm [109]. However, in a recent study by Zhang et al., glutathione-coated AuNPs with sizes less than 2 nm have the ability to accumulate preferentially within subcutaneous tumor-bearing mice providing strong radioenhancement for cancer therapy [111]. More recently, Joh et al. showed that PEG-AuNPs and radiation therapy can enhance DNA damage and tumor cell destruction and improve survival in mice with orthotopic glioblastoma multiforme tumors [107]. Intriguingly, they also showed that ionizing radiation could compromise tumor vasculature significantly increasing the accumulation of AuNPs within brain tumor-bearing mice. All of these strategies mentioned are examples of passive tumor targeting of AuNPs that are reliant on the EPR effect. To our knowledge, a study conducted by Chattopadhyay and coworkers is the only one that has assessed the in vivo radioenhancement effects of targeted AuNPs, using a tumor-specific HER-2-targeted nanoplatform [101]. However, the benefits of having targeted AuNPs versus untargeted were not conclusive as there were no in vivo comparisons made, and AuNPs were administered via intratumoral injections.
Table 10.2
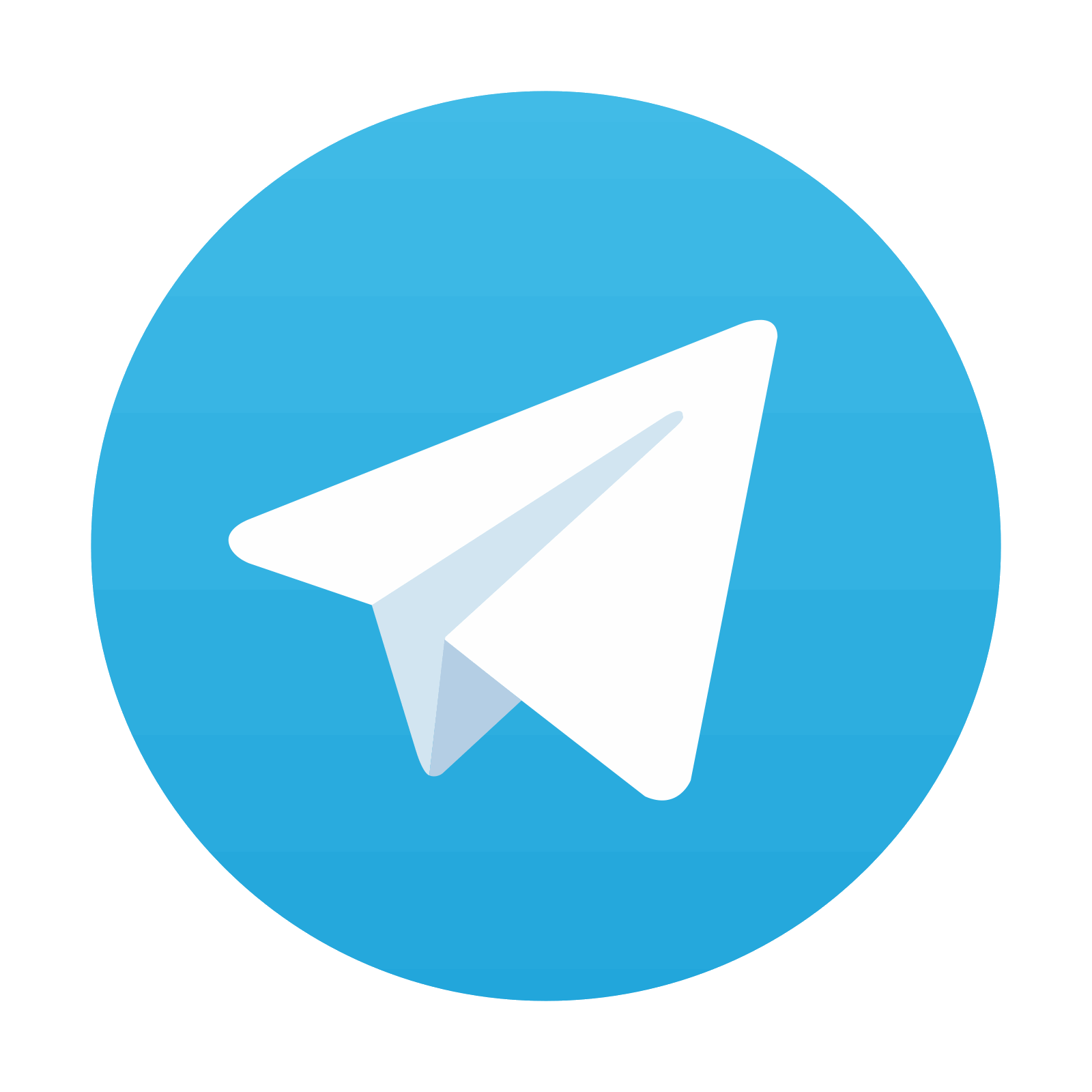
Summary of in vivo radiosensitization experiments using AuNPs
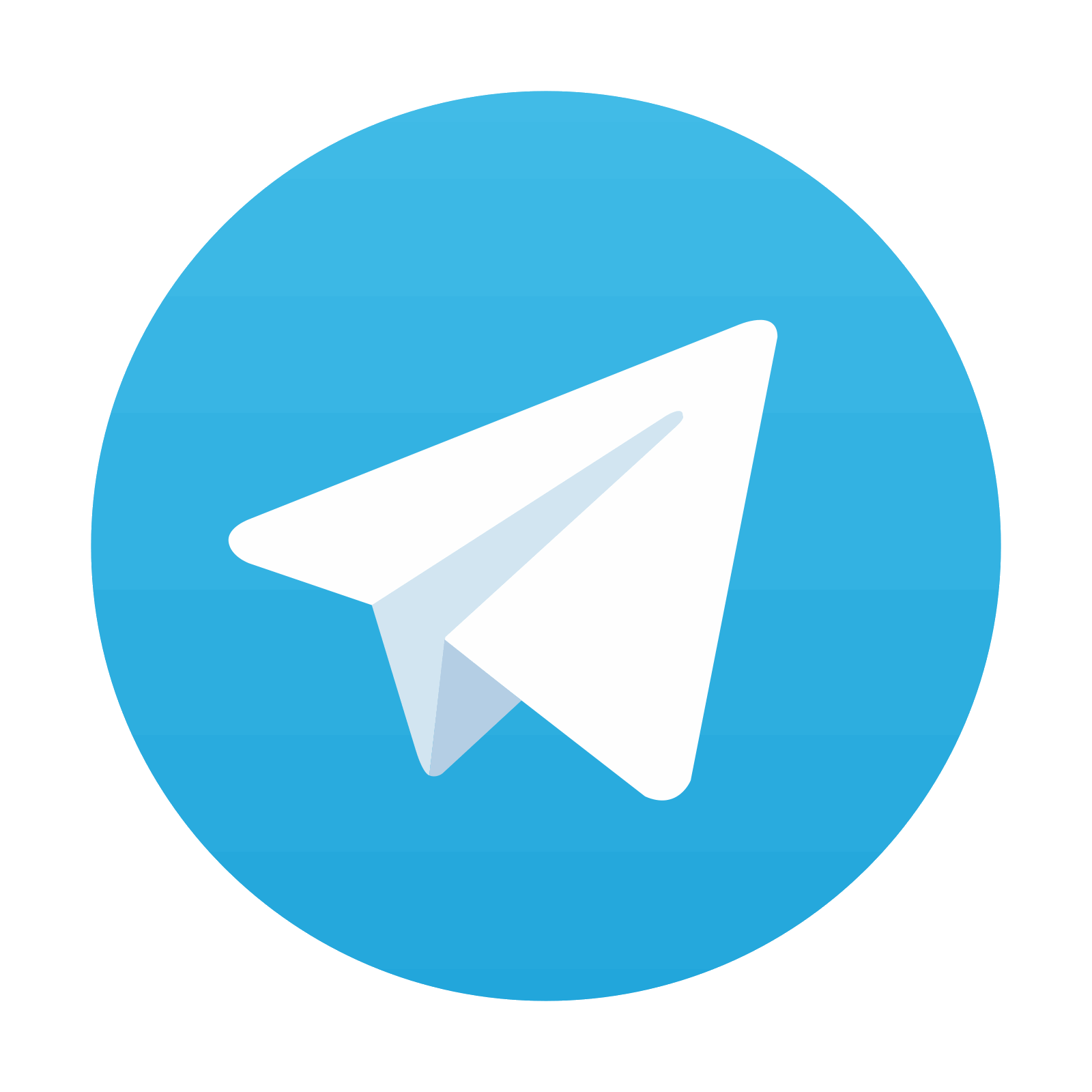
Stay updated, free articles. Join our Telegram channel
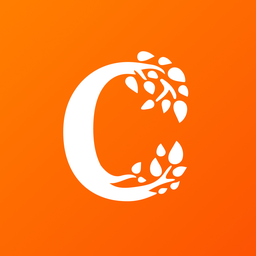
Full access? Get Clinical Tree
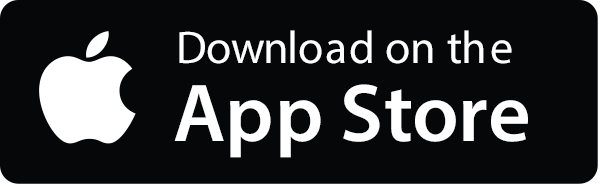
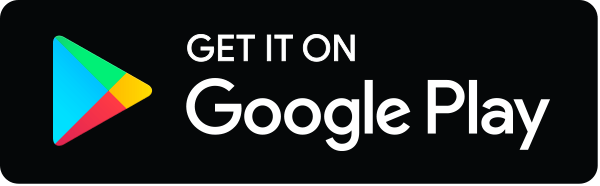
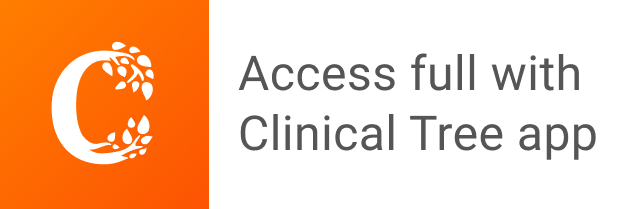