(1)
Radiation Monitoring Devices Research Nuclear Medicine, Water town, Massachusetts, USA
9.1 Introduction
9.3.1 Generator
9.3.2 Reactor
9.3.3 Cyclotron
9.4 Radiation Dose
9.5.2 Proton Beam Therapy
9.5.3 Neutron Beam Therapy
9.6.2 Gamma Knife
9.6.3 Proton Beam
9.7 Scintigraphy
9.7.1 Angiography (CTA, MRA)
9.7.2 CT
9.7.3 PET
9.9 Final Remarks
Abstract
Nuclear medicine is a subspecial branch of radiology, which utilizes radioactive materials to create images of a body anatomy and its function. The Wikipedia encyclopedia defines nuclear medicine as the medical specialty involving diagnostic tests and therapeutic examinations. Therapeutic examinations are performed with unsealed sources consisting of radionuclides (shown in Table 9.1) or radiopharmaceuticals labeled as radionuclides (radiopharmaceuticals) [1].
9.1 Introduction
Nuclear medicine is a subspecial branch of radiology, which utilizes radioactive materials to create images of a body anatomy and its function. The Wikipedia encyclopedia defines nuclear medicine as the medical specialty involving diagnostic tests and therapeutic examinations. Therapeutic examinations are performed with unsealed sources consisting of radionuclides (shown in Table 9.1) or radiopharmaceuticals labeled as radionuclides (radiopharmaceuticals) [1].
Table 9.1
Radionuclides administered for most frequently performed procedures
Activities | Radionuclide | Chemical form | Dose (MBq) |
---|---|---|---|
Bone scan (planar 98 %) | 99mTc | Phosphates | 400–775 |
SPECT (2 %) | 99mTc | 500–800 | |
Lung perfusion (planar (99.99 %) | 99mTc | MAA (macroaggregated albumin) | 50–200 |
SPECT (0.01 %) | 100–100 | ||
Myocardium (SPECT 98 %) | 99mTc | Tetrofosmin | 250–600 |
Planar (2 %) | 99mTc | Tetrofosmin | 370–400 |
Lung ventilation | 81mKr | Gas | – |
Kidney | 99mTc | MAG3 | 20–200 |
Kidney | 99mTc | DMSA | 23–200 |
GFR measurement | 99mTc | EDTA | 0.2–4 |
Myocardium (SPECT 87 %) | 99mTc | Sestamibi | 388–450 |
Planar (13 %) | 99mTc | Sestamibi | 400–500 |
Lung ventilation | 99mTc | DTPA (diethylenetriamine pentaacetic acid) | 10–2,500 |
Myocardium (SPECT 98 %) | 201Tl | Thallous chloride | 55–80 |
Planar (2 %) | 201Tl | Thallous chloride | 78–80 |
Lung ventilation | 99mTc | Technigas | 15–300 |
Thyroid | 99mTc | Pertechnetate | 35–180 |
Thyrotoxicosis therapy | 131I | Iodide | 185–800 |
Cardiac blood pool | 99mTc | Normal erythrocytes | 370–800 |
Tumors (PET) | 18 F | FDG (fluorodeoxyglucose) | 222–400 |
Infection, inflammation, tumors | 99mTc | Exametazime | 40–600 |
Helicobacter pylori test | 14C | Urea | 0.01–0.2 |
Kidney | 99mTc | DTPA | 12–800 |
Lung ventilation | 133Xe | Gas | 200–600 |
Cerebral blood flow (SPECT 94 %) | 99mTc | Exametazime | 72–800 |
Planar 6 % | 99mTc | Exametazime | 200–500 |
Radiopharmaceuticals introduced inside a patient’s body mostly have two components: a radionuclide, an excited state of atom which emits energy so that the atom can convert to a stable form and a carrier molecule that travels through the body until it interacts with its target cell tissue or organ system. For example, highly lipophilic molecules (such as exametazime) that penetrate the protective blood brain carrier are taken up in brain tissues. Since uptake will be proportional to regional blood flow, the amount of tracer deposited will be indicative of how much blood flow reaches a particular area. Figure 9.1 shows the picture of a brain taken after 99mTc (Tc is technetium, its half-life is 6 h, and the end point energy is 0.292 Me, used to image skeleton, heart muscle, brain, thyroid, lungs, liver, spleen, kidney, gall bladder, bone marrow, salivary and lacrimal glands) is administered into the body [2, 3].
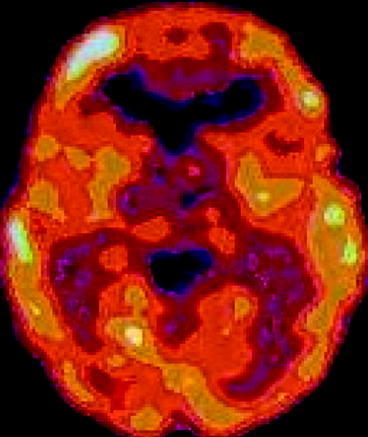
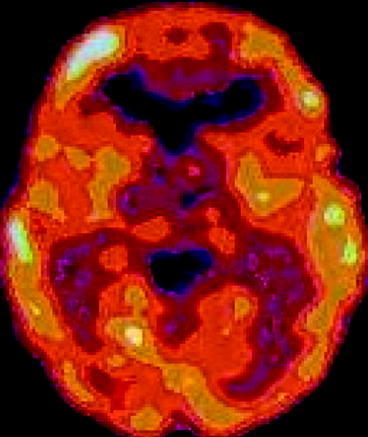
Fig. 9.1
99mTc exametazime brain imaging (Courtesy, Purdue University, IN)
The characteristics of an ideal radioisotope for therapy are nontoxic, and they are administered in very low concentrations. Not only that, they need careful scrutiny, quite different from those required for imaging. For imaging, the energy of the radioisotopes is deposited in the camera crystal, without significant absorption in the tissue, whereas the energy of the therapeutic radioisotope must be deposited in the tissue to damage the DNA (deoxyribonucleic acid) chains to keep the diseased cells from replicating [4]. DNA plays an important role in cellular multiplication and function, and ionizing radiation can cause deletions or substitutions of bases and/or actual breaks in DNA chains [5].
Unlike magnetic resonance imaging (MRI) [6, 7] and computer tomography (CT), nuclear medicine uniquely provides information about both the structure and the function of body organs within the body. A large portion of the diagnostic work involves imaging, and a small portion of the diagnostic work involves non-imaging parts (the assay of blood samples to measure the glomerular filtration of the kidneys).
No single isotope dominates in radioisotope therapy as 99mTc does in nuclear imaging. The design of a successful therapeutic radiopharmaceutical requires (a) selection of the targeting molecule to deliver the radioisotope to the diseased site properly, (b) accurate calculations of the amount of the dose to destroy the affected cells [8], and (c) development of a method for destroying the bad cells without adversely affecting the good cells. The widespread availability of the radioisotope’s therapy depends upon the availability of the therapeutic doses, appropriate legends chemistry techniques, emitters at reasonable costs, and long-term therapy without complications such as bone marrow toxicity and renal damage [9].
9.2 Administration of the Radionuclides
These radionuclides used in nuclear medicine are administrated into the body either by injection or aggregate form according to the parts of the body or organ that will be studied through the medical imaging [10, 11]. For example, intravenous, subcutaneous is used to investigate the lymphatic system, intrasynovial to take the image of the knee joint, inhalation in gaseous form, like krypton-81 (81Kr), for investigating the function of lungs, ingestion (99Tc added to scrambled eggs) to investigate gastric emptying, or topical application of the radiopharmaceutical directly to the area to be investigated (e.g., 99Tc eye drops to investigate tear duct flow). Depending upon on which type of scan is being performed, the imaging will be done immediately, a few hours later, or even several days after the radiopharmaceutical agent is administered. Figure 9.2 shows the process sequence of the medical imaging and the administration of the radioisotope inside the region of interest (ROI).
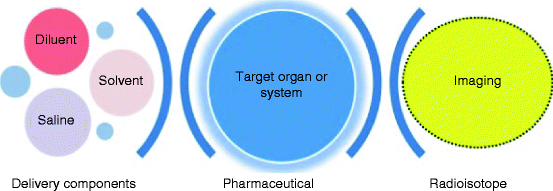
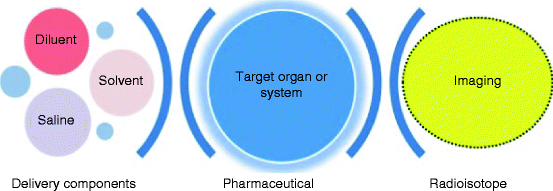
Fig. 9.2
Steps showing the final imaging of the embedded radioisotope and the administration of radionuclides
Specifically, nuclear medicine can be used to:
(a)
Analyze kidney function
(b)
Image blood flow and function of the heart
(c)
Scan the lungs for respiratory and blood-flow problems
(d)
Identify blockage of the gall bladder
(e)
Locate the presence of infection
(f)
Identify bleeding of the bowel
(g)
Measure thyroid function to detect an overactive or underactive thyroid
9.3 Preparation of Radionuclides
Most of the radionuclides are made either by bombarding stable atoms or by splitting massive atoms. There are three types of equipment commonly used to introduce radionuclides, namely, generators, cyclotron, and nuclear reactors.
9.3.1 Generator
The most commonly used equipment, which is used to make nuclear medicine, is the generator. The most commonly used nuclides used in nuclear medicine is 99 m-Tc (99mTc) which is produced in a highly shielded column of fission product Mo-99 (99Mo-parent) bound to alumina (Al2O3). The 99Tc (daughter) is milked (eluted) by drawing sterile saline through the column into the vacuum vile. The parent 99Mo is firmly bound to alumina, and as a result, the eluted 99mTc contains negligible amounts of 99Mo, and the daughter 99Tc, which is washed away with saline, is collected in a vile (vacuum). From the sterile solution containing 99mTc, sodium pertechnetate injection is prepared for applications into the body organs and remains on the column. The generator is called a cow; the daughter nuclide is referred to as milking and surrounding lead shield is called a pig (Fig. 9.3). The 99mTc decays to 99Tc during radioactive decays with principal radiation γ-ray, having specific γ-ray constant of 0.19 mGy per MBq-h-1at-1 cm. Figure 9.3 shows a cross-sectional view of the 90Mo–99mTc generator.
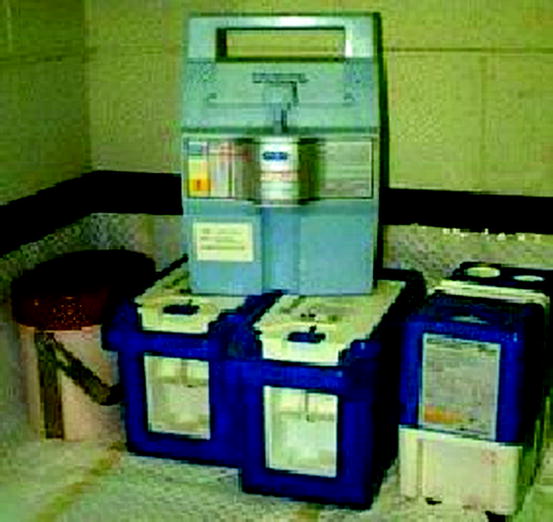
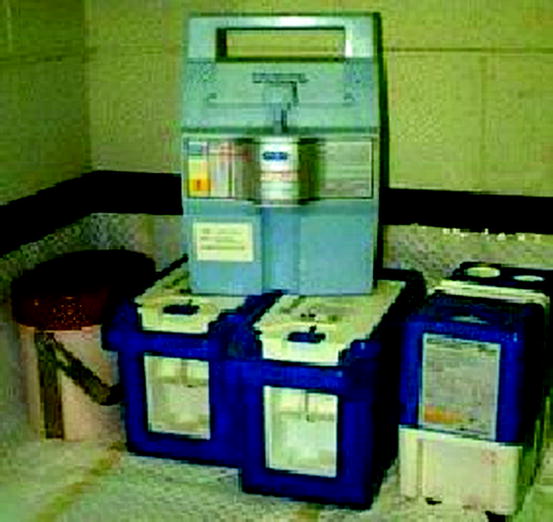
Fig. 9.3
Cross-sectional view of 99mTc generator (Courtesy: WIKI)
9.3.2 Reactor
Radionuclides for the use in nuclear medicine are also produced in nuclear reactor by the process of fission, neutron capture, or transmutation, for example, 131I, 133Xe, and 99Mo. Table 9.2 shows the parent and daughter nuclei and their applications, and the isotopes used in nuclear medicine are given in Table 9.3. Atomic nuclei with the same number of protons, but with different numbers of neutrons, are called isotopes. Isotopes are identified as stable, when the protons and the neutrons in the atom can coexist in a state of peaceful tranquility. On the other hand, the isotopes that spontaneously emit radiation are called radioisotopes. The relative abundance of three isotopes of potassium (K) (39K, 40K, and 41K, have 19 protons, and (39–19), (40–19), and (41–19) neutrons) are constant, regardless of the source. Even the human body contains almost 150–200 g of potassium (K) of which 20–25 mg exists as a radioisotope (40K). The other natural radioisotopes are carbon (14C) and hydrogen (3H, tritium) that result from the nuclear reactions of the atmospheric nitrogen (N). During our lifetime, we absorb and excrete 14C, and as a result, 14C levels in our tissues gradually increases to an equilibrium level and in course of time it decays due to its radioactive nature. But the half-life of 14C is 5,730 years, so the effect of decay is not much noticeable during our lifetime.
Table 9.2
Splitting of the nucleus (Parent–daughter relation inside generator)
Parent | Daughter | Applications |
---|---|---|
99Mo | 99mTc | Radiopharmaceuticals |
82Sr | 82Rb | Cardiac perfusion imaging |
81Rb | 81mKr | Lung ventilation scans |
Table 9.3
Radioisotopes used in nuclear medicine
Radioisotopes | Half-life | Application |
---|---|---|
Bismuth (Bi)-213 (213Bi) | 46 min | Used for targeted alpha therapy (TAT) |
Chromium-51 (51Cr) | 28 days | Use to level red blood cells and quantify gastrointestinal protein loss |
Cobalt-60 (60Co) | 10.5 months | External beam therapy (EBT) |
Copper-64 (64Cu) | 13 h | Study genetic diseases affecting copper metabolism |
Dysprosium-165 (165Dy) | 2 h | Used as an aggregated hydroxide for arthritis |
Erbium-169 (169Er) | 9.4 days | Arthritis joints |
Holmium-166 (166Ho) | 26 h | For diagnosis and treatment of liver tumors |
Iodine-125 (125I) | 60 days | For cancer brachytherapy, diagnoses of the filtrate rate of kidneys, deep vein thrombosis in leg, and radioimmunoassays |
Iodine-131 (131I) | 8 days | Thyroid cancer, liver, renal blood flow, and urinary obstruction |
Iridium-192 (192Ir) | 74 days | Used as wire in internal radiotherapy and cancer treatment |
Iron-59 (59Fe) | 46 days | To study iron metabolism in spleen |
Lutetium-177 (177Lu) | 6.7 days | For small tumors |
Molybdenum-99 (99Mo) | 66 h | Used as parent in a generator to produce 99mTc |
Palladium-103 (103Pd) | 17 days | Prostate cancer |
Phosphorus-32 (32P) | 14 days | Treatment of excess red blood cells (polycythemia vera) |
Potassium-42 (42K) | 12 h | To determine potassium in coronary blood flow |
Rhenium-186 (186Re) | 17 h | Pain relief in bone cancer |
Samarium-153 (143Sm) | 47 h | Treatment in bone, prostate, and breast cancer (Quadramet) |
Selenium-75 (75Se) | 120 days | To study production of digestive enzymes (selenomethionine) |
Sodium-24 (24Na) | 15 h | To study electrolysis within the body |
Strontium-89 (89Sr) | 50 days | Pain reliever for prostate and bone cancer |
Technetium-99 (99mTc) | 6 h | Used to image the skeleton, heart muscle, brain, thyroid, lungs, liver, spleen, kidney, gall bladder, bone marrow, salivary, and lacrimal glands |
Xenon-133 (133Xe) | 5 days | To study pulmonary (lung) ventilation |
Ytterbium-169 (169Yb) | 32 days | To study cerebrospinal fluid in the brain |
Ytterbium-177 (177Yb) | 1.9 h | Progenitor of Lu-177 |
Yttrium-90 (90Y) | 64 days | Used for cancer brachytherapy and pain reliever in arthritis |
A revolution in radiotherapy has been possible for the reactor-based radionuclides, which are β-emitters such as 153 Sm, 186Re, 166Ho, 177Lu, and 105Rh. These radionuclides are used in more sophisticated radioactive targets including radioactive intra-arterial microspheres, chemically guided bone agents, labeled monoclonal antibodies, and isotopically tagged polypeptide receptor-binding agents [12]. Figure 9.4 shows the picture of the inside of a nuclear reactor.
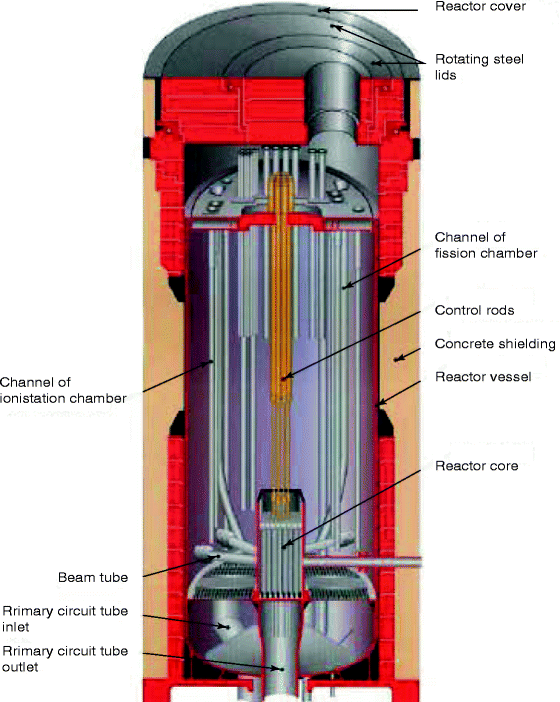
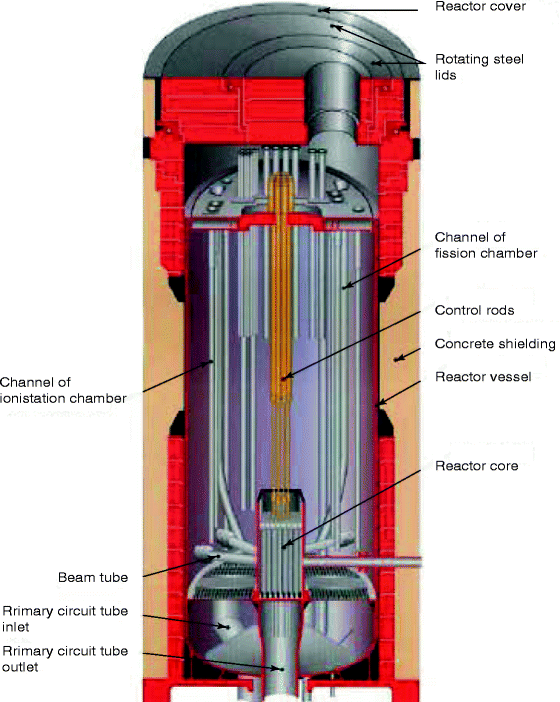
Fig. 9.4
An LVR-15 light-water moderated and cooled tank nuclear reactor with forced cooling (Photo courtesy UJV)
9.3.3 Cyclotron
Ernest Lawrence in Berkeley, CA, reported the invention of cyclotron in 1934 and recognized the possibilities of the machine to produce artificial radioisotopes for the application in medicine. In 1937, Joseph Hamilton was the first to use the radioisotopes to study circulatory physiology [13]. The cyclotron accelerates charged particles such as hydrogen nuclei (protons) and heavy hydrogen nuclei (deuterons) to high energies. The molecules of deuterium at the center of the cyclotron are bombarded with electrons whose energy is high enough to produce positive ions during collisions.
Figure 9.5 shows the schematic of a cyclotron. D1 and D2 are the two dees made of copper sheet to which a high-frequency oscillator applies the accelerating voltage. The direction of the potential difference across the gap between the dees is made to change signs some millions of times per second. The dees are immersed in a magnetic field produced by a large electromagnet, and the space where the ions will move is evacuated to avoid collisions between ions. Table 9.4 shows some of the radioisotopes produced in cyclotron.
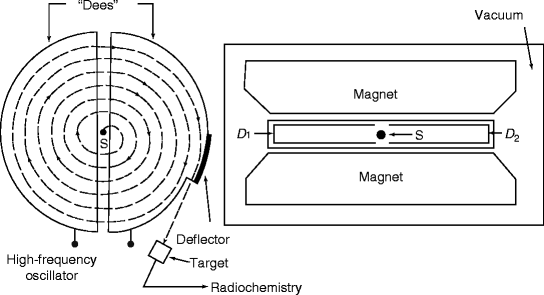
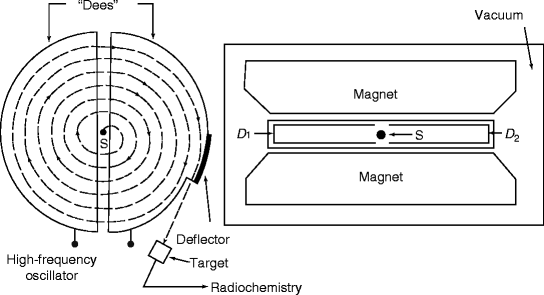
Fig. 9.5
Schematic representation of a cyclotron
Table 9.4
Isotopes produced in cyclotron
Radioisotopes | Half-life | Application |
---|---|---|
Carbon-11 (11C) | 20.4 min | Used in PET as positron emitters to study neuropharmacology and dementia |
Cobalt-57 (57Co) | 272 h | Used as a marker to estimate organ size |
Fluorine-18 (18F) | 109.8 min | Used in PET as positron emitters to detect cancers cells |
Gallium-67 (67Ga) | 78 h | For tumor imaging and location of inflammatory lesions |
Indium-111 (111In) | 2.8 h | Used for diagnostic studies of the brain, infection, and colon transit |
Iodine-123 (123I) | 13 h | Used in the diagnosis of thyroid function |
Krypton-81m (81Krm) | 13 s | To image pulmonary ventilation for asthmatic patients and lung disease |
Nitrogen-13 (13N) | 10 min | Used in PET as positron emitters to study neuropharmacology and dementia |
Oxygen-15 (15O) | 2 min | Used in PET as positron emitters to study neuropharmacology and dementia |
Rubidium-82 (82Rb) | 65 h | To image myocardial perfusion |
Strontium-92 (92 Sr) | 65 h | Used as parent in a generator to produce Rb-82 |
Thallium-201 (201Th) | 73 h | To diagnostic studies of coronary artery disease and location of lymphomas |
9.4 Radiation Dose
The direct measurement of photons (X–ray, gamma rays, or bremsstrahlung, beta particles or alpha particles) is often the most accurate method to determine the quantity and location of radioactive material in the human body. The activity of a radioisotope is defined as its rate of decay, {(dN/dt) = (λN)}, where N is the number of radioactive nuclei, t is the time to decay, and λ is the decay constant. The most commonly used unit for radioactivity is expressed in curie (Ci), which means 3.7 × 1010 disintegrations per second. However, the SI unit of radioactive dose is becquerel (Bq).
A patient undergoing a therapy is administered a dose of the nuclear medicine which can range from 0.006 mSv (millisieverts) for a 3 MBq (megabecquerels) of chromium (Cr)-51 (EDTA measurement of glomerular filtration rate) to 37 mSv for a 150 MBq of thallium (Tl)-201 for nonspecific tumor imaging. However, for bone scan with 600 MBq of Tc-99 m-MDP, the effective dose is 3 mSv [14, 15].
9.5 Radiation Therapy
Radiation therapies that are most frequently performed in nuclear medicine can be categorized as (Radiological Society of North America Inc., RSNA):
1.
Brain tumors
2.
Breast cancer
3.
Head and neck cancer
4.
Lung cancer
5.
Prostate cancer
The procedure to cure these cancers is done by exposing the cancerous cells by external radiation by adopting any one of the therapies according to the suitability such as (a) external beam therapy (EBT), (b) intensity–modulated radiation therapy (IMRT), or (c) stereotactic radiosurgery (SRS).
9.5.1 External Beam Therapy (EBT)
In external beam therapy (EBT), high energetic X-ray is administered to the location of the affected cells. The beam is generated outside the patient’s body usually by either a linear accelerator (LINC), orthovoltage X–ray machines, Cobalt–60 machines, proton beam machines, or neutron beam machines. In EBT no radioactive sources are placed inside the patient’s body. EBT can be successfully applied to treat any of the following diseases such as (1) breast cancer, (2) colorectal cancer, (3) head and neck cancer, (4) lung cancer, and (5) prostate cancer as well as many other cancerous cells inside a specific area of the body.
9.5.2 Proton Beam Therapy
It is a form of external beam radiation treatment that uses protons rather than X-rays to treat certain types of cancer and other diseases. The physical characteristics of the proton therapy beam allow doctors to more effectively reduce the radiation dose close to nearby healthy tissues.
9.5.3 Neutron Beam Therapy
It is a specialized form of external radiation, which consists of neutrons instead of X-rays. Neutron beam therapy is a powerful tool, which is used to treat certain types of tumors that are radiation resistant, meaning that they are difficult to kill by using ordinary X-ray or proton beam therapy. As a matter of fact, neutrons have a greater biological impact on cells than other types of radiation.
9.6 Intensity-Modulated Radiation Therapy (IMRT)
In intensity-modulated radiation therapy (IMRT), a computer-controlled X-ray accelerator is used to deliver a precise radiation dose to a malignant tumor or to the specific areas of a cancerous cell [16, 17]. The radiation dose is designed to conform to the three-dimensional (3-D) shape of the tumor by modulating or controlling the intensity of the radiation beam [18]. The equipments that are very frequently used to administer the radiation from outside are (1) linear accelerator (LINAC), (2) Gamma Knife, and (3) proton beam. In IMRT, the ratio of normal tissue dose to tumor dose is reduced to a minimum. As a result, higher and more effective radiation doses can safely be delivered to tumors. Moreover, it reduces the treatment toxicity even when the doses are not increased. The most important of all is that the patterning of the radiation allows doctors to deliver radiation to different places in the body (e.g., paranasal sinuses) that have been difficult with other external radiation therapy.
9.6.1 Linear Accelerator (LINC)
LINC is a device mostly used for external beam radiation treatments for cancer patients [19]. In linear accelerator (LINC), electrons are accelerated by microwave technology similar to that used in radar. The waveguide then allows these electrons to collide with a heavy metal target. A portion of these X-rays coming out of the accelerator (called gantry) are collected and shaped into a narrow beam. The beam is usually rotated around the affected area of the cancerous cells of the body (Fig. 9.6).
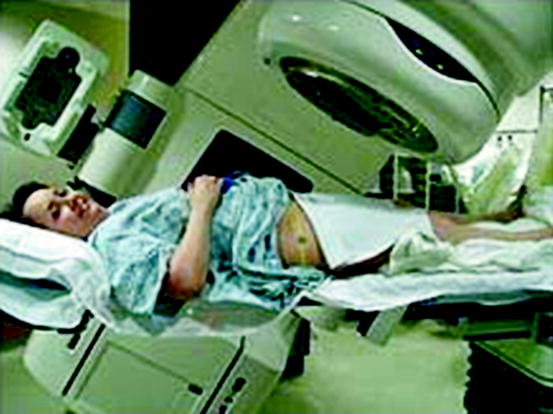
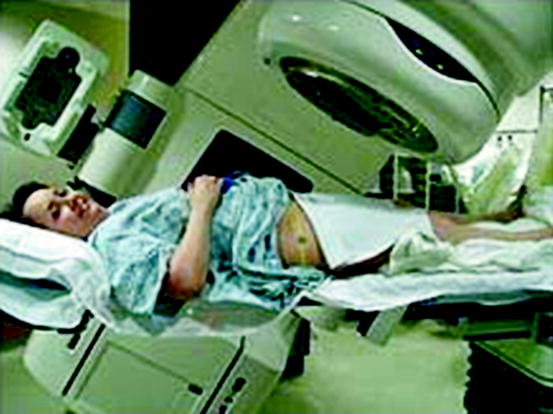
Fig. 9.6
Picture of a linear accelerator in use for external beam therapy (Courtesy:WIKI)
The safety of the patient is very important during treatment, and the radiotherapist should continuously monitor the patient through a closed-circuit television monitor [20]. The development of a compact and fully digital LINC provides beam accuracy, treatment automation, and patient comfort—translating maximum radiation dose to the tumor, minimum dose to the surrounding tissue, and less time on table for the patients. Modern radiation machines have internal checking systems to provide further safety so that the machine will not turn on until all the treatment requirements are met.
9.6.2 Gamma Knife
The Leksell Gamma Knife is a neurosurgical device used to treat mostly brain tumors with radiation therapy. The device was invented by Lars Leksell, a Swedish neurosurgeon, in 1967 at the Karolinska Institute in Sweden. The procedure involves a highly targeted radiation source, which is focused precisely on the target from many different directions, and it has brought a revolutionary type of surgery in the field of nuclear medicine. The individual radiation beam is weak enough to harm the brain tissue it passes through. The beams of the gamma-ray radiation called blades are programed to target the lesion at the point where they intersect. The exposure session is generally brief and precise. Thus, the Gamma Knife is able to cut deep into the brain without using a scalpel at all.
Gamma Knife surgery is preferred to noninvasive (non-incision) surgery for brain tumors, arteriovenous malformations, and brain dysfunction like neuralgia [21]. It is an alternative nonsurgical operation for many patients for whom the traditional surgery is not an option. The greatest advantage of the Gamma Knife technology is that it allows treatment of inoperable lesions. The risks of Gamma Knife radiosurgery treatment include but are not limited to radiation necrosis, secondary malignancy head frame, paralysis, and death.
Figure 9.7 shows the picture of a Gamma Knife setup showing in details of the machine with the radiation source which can be focused from all directions. The setup also includes computerized data from imaging tests, to pinpoint areas within the brain and destroy them using multiple beams of gamma radiation [22]. Cumulative effect of the gamma rays from all directions produces a high dose of radiation at the exact site of the lesion, without damaging the healthy cells.
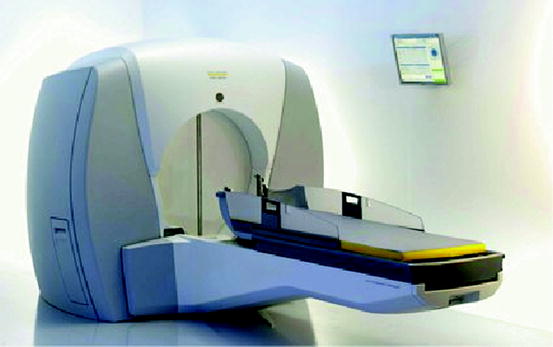
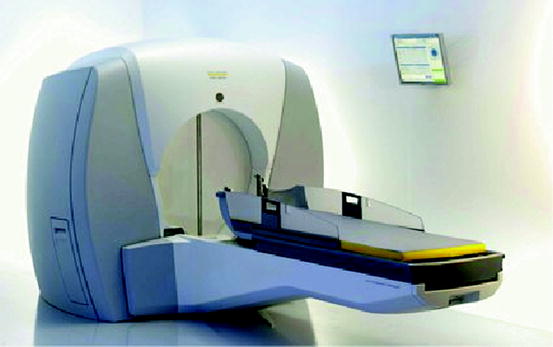
Fig. 9.7
The detailed drawing of the Gamma Knife (Courtesy: Virginia Med., VA)
9.6.3 Proton Beam
The proton beam therapy or radiosurgery has properties different from the radiation used by Gamma Knife and LINAC machines. Proton beams deliver almost all their radiation at a set distance from the radiation source, rather than gradually releasing the energy as the beam travels. This difference eliminates the need for multiple beams from different directions as we have seen in Gamma Knife operation. To improve precision, a head frame is used and metallic beads are also inserted under the skin to help the computer triangulate tumor volume. Sometimes, the instrument might have the facility to rotate the body so that the proton beam can be focused on the tumor from different angles. The main drawback with the process is that it required a highly specialized particle accelerator called synchrocyclotron, which made the processing costly [23].
Proton radiation therapy is considered as one of the most precise forms of noninvasive image-guided cancer therapy [24]. It is based on the well-defined range of protons in material, with low entrance dose and a maximum (Bragg peak) and a rapid distal dose falloff, providing better sporting of healthy tissue and allowing higher tumor doses than conventional radiation therapy with photons [25, 26].
9.6.4 Stereotactic Radiosurgery (SRS)
Stereotactic radiosurgery (SRS) is a nonsurgical procedure that applies radiation therapy in a highly precise form to treat brain tumors and other abnormalities of the brain. The radiosurgery in SRS has such a dramatic effect in the target zone that the changes are considered as surgical [27]. The treatment involves the delivery of a single high dose or smaller multiple doses of radiation from an external source such as (a) linear accelerator or cyclotron, (b) Gamma Knife, and (c) proton beam. The SRS does not remove the tumor; instead, it destroys the DNA of tumor cells. It provides an external frame of reference for the subsequent radiation treatment planning. The linear accelerator, the cobalt source machines, and proton radiosurgeries are three different types of machines generally used in SRS.
9.7 Scintigraphy
The second phase of the test is the medical imaging with the help of a detector. It is referred to as radionuclide imaging or nuclear scintigraphy [28, 29]. In diagnosis, radioactive substance is administered to a patient, and the radiation emitted from the radionuclide is measured. The majority of these diagnostic tests are performed with gamma camera. In diagnostic radiology, imaging of the affected tissues has brought revolution in the detection of the affected diseased cells. In diagnostic radiology the imaging technologies are different according to the way the procedures are followed. These are:
1.
Angiography
2.
Computed tomography (CT)
3.
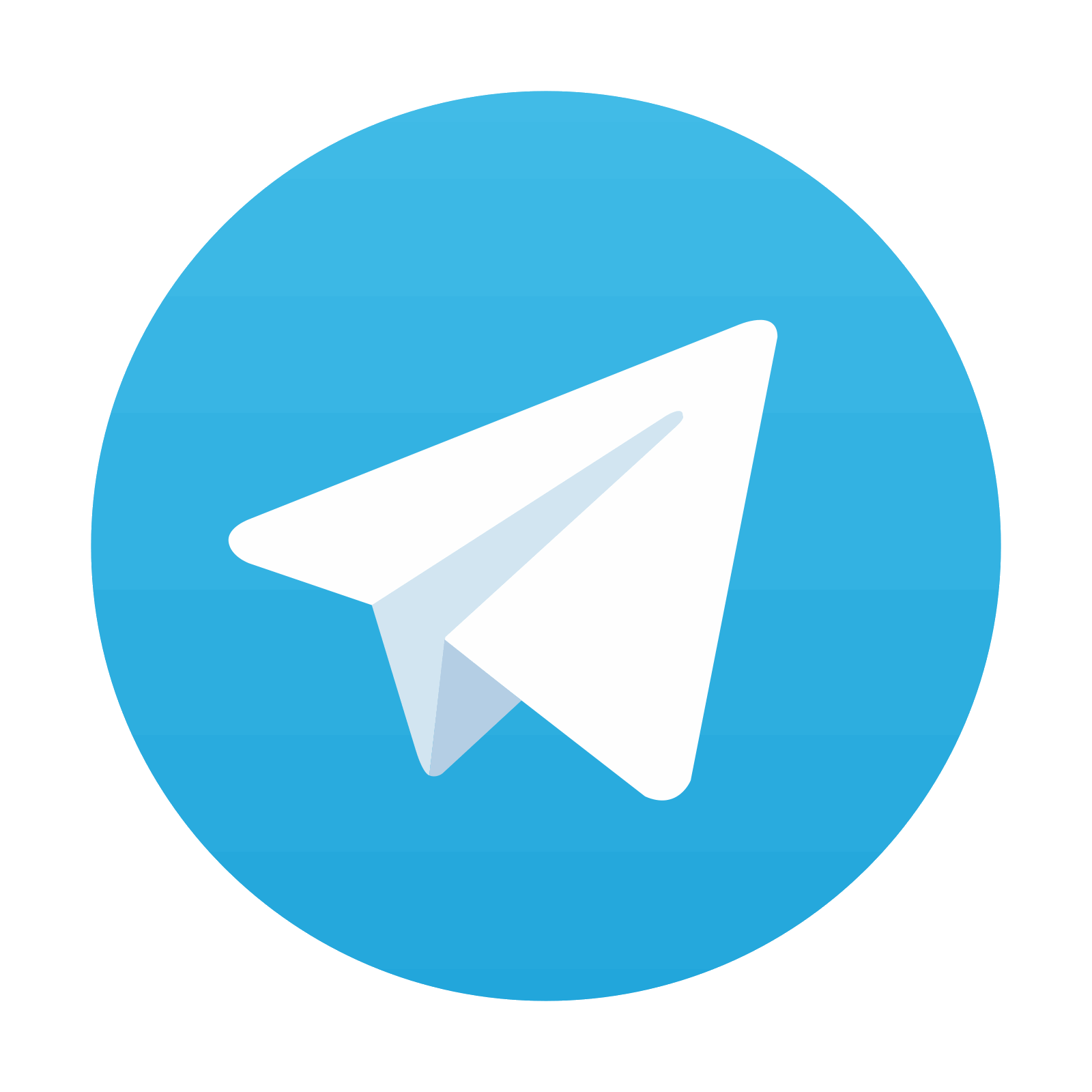
Positron emission tomography (PET)
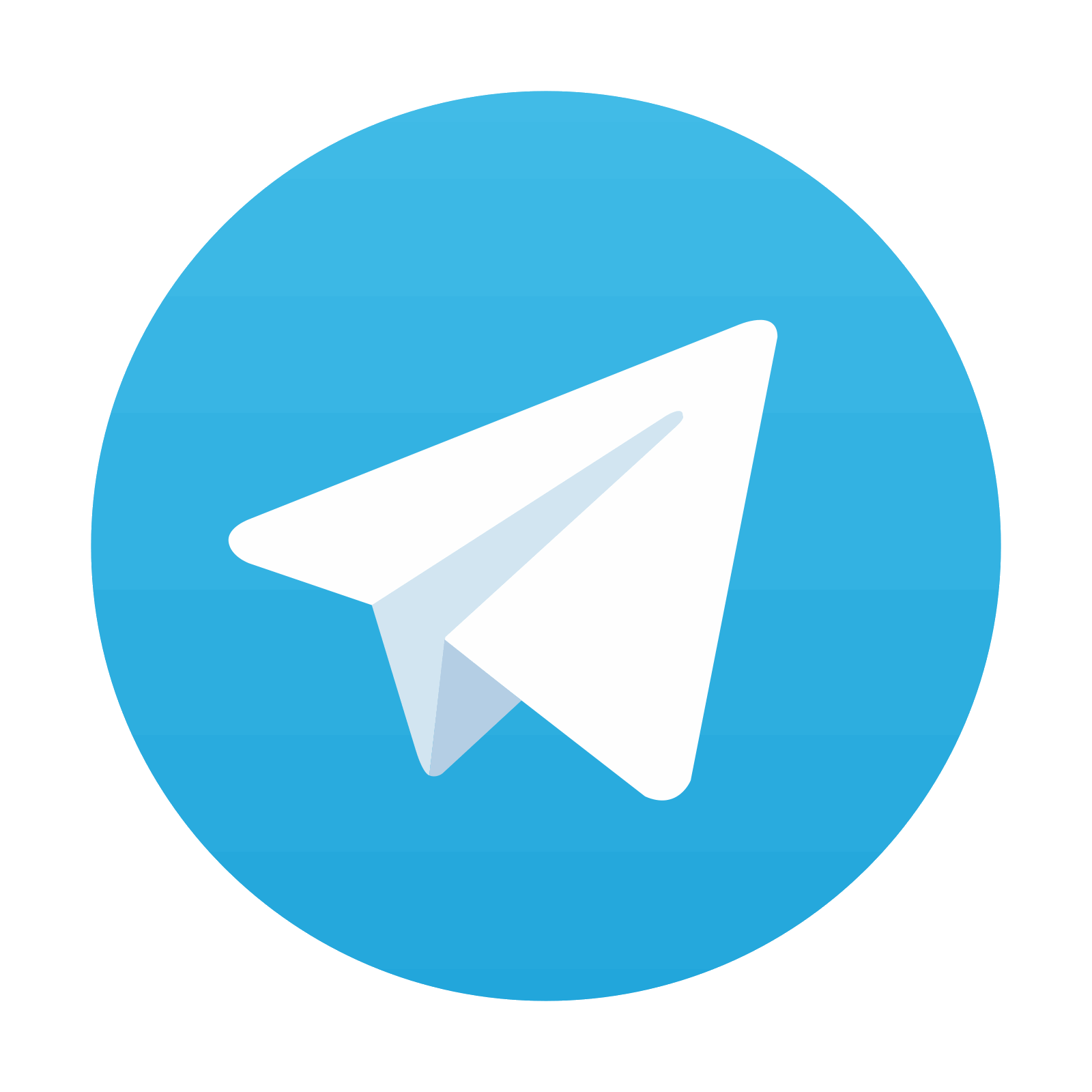
Stay updated, free articles. Join our Telegram channel
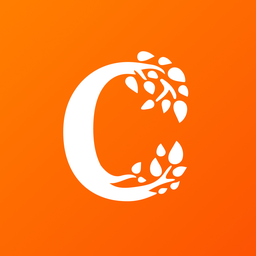
Full access? Get Clinical Tree
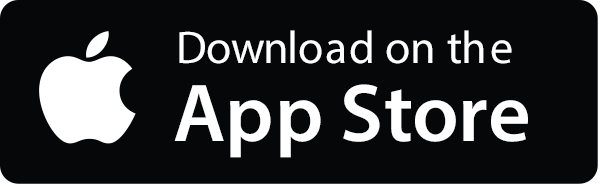
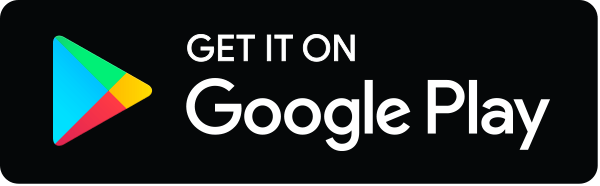
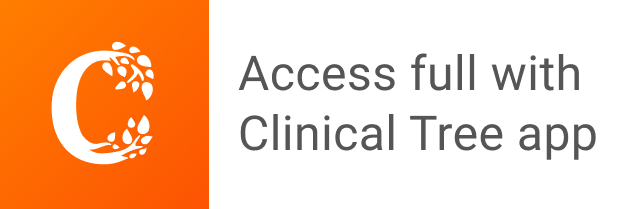