Bruno QUESSON1,2 and Valéry OZENNE1 1 CRMSB, CNRS, Université de Bordeaux, France 2 IHU Liryc, Université de Bordeaux, France The goal of interventional MRI is to provide visual real-time feedback to the personnel performing therapeutical interventions (puncture, surgery, thermal ablation, radiotherapy, etc.) via a medical therapeutic device (MTD). It is employed in several medical fields (neurology, oncology, urology, cardiology, etc.). Many imaging modalities (X-rays, ultrasounds, pre-operative optical imaging and MRI) can be exploited, with the choice dependent on several aspects (targeted application, access to instrumentation, type of information sought in the images, equipment cost and compatibility with the MTD). Regardless of the application and imaging modality, the procedures typically include the steps listed below. In this pre-interventional phase, the goal is to define the main steps of the intervention that include (i) delimiting the location to be reached and/or the region to be treated via the MTD and critical adjacent tissues to be preserved; (ii) the choice of the access path: percutaneous, vascular or non-invasive; and (iii) the choice of the MTD and its interventional mode. For this purpose, a map of the subject’s anatomy (ideally in 3D) that covers the entire region penetrated by the MTD is usually obtained before the treatment. This map can be an anatomical image, a 3D model (e.g. a 3D mesh) or a functional representation (e.g. a 3D map of the electrical cardiac activity). Such pieces of information can be acquired several days before the intervention or at the very beginning of the interventional session if the imaging device makes it possible. This phase requires real-time imaging to interactively guide the intervention by combining live images with data obtained during the planning phase. This sets a minimum image acquisition rate for a smooth and, ideally, latency-free display of relevant information to the person performing the intervention. At first, the MTD is typically moved (ballistically) toward the area to be treated, and then the intervention is performed. Ideally, these steps are guided via real-time imaging. However, if this is impossible (e.g. if the device cannot be operated during image acquisition), intermittent imaging is performed, for example, by iteratively re-positioning the therapeutic device at the target area. Once the therapeutic intervention has been performed, one or several imaging modalities can be used to evaluate the treatment effect. Such post-operative imaging can be performed immediately after the intervention and/or several weeks later, if necessary. Table 11.1 lists the different imaging modalities for each intervention step. Table 11.1. Comparison of the performance of different imaging modalities: ++: excellent; +: acceptable; –: poor MRI presents undeniable advantages for several procedural steps. However, the issues of costs and instrumentation accessibility have so far hindered the clinical development of interventional MRI in favor of other imaging modalities such as ultrasound and X-ray scans or fluoroscopy. On the other hand, a certain number of technical barriers related to the acquisition and image reconstruction techniques, hardware of medical devices that are used in highly magnetic environments, and numerical computation capabilities have also challenged the growth of this discipline. Nevertheless, all these obstacles are getting tackled, paving the way for interventional MRI applications with significant potential. Figure 11.1 provides a schematic representation of an MTD guided via MRI. Figure 11.1. Schematic representation of an interventional MRI device. (1) MRI provides images during the therapeutic procedure. (2) These images are analyzed and visualized in real time via software that eventually interacts with the MTD (3) to optimize the therapy Before continuing, the notion of real-time imaging has to be defined. It would not make any sense to provide absolute values of imaging rate in seconds or minutes. In fact, the rate strongly depends on the application and type of information that has to be obtained. The notion of real-time imaging has to be put in perspective with the characteristic time of the biological phenomenon that has to be investigated, the specific constraints related to the foreseen treatment and the targeted organ. To illustrate this point, let us consider the example of thermal radiofrequency therapies that are commonly used to treat liver tumors (via percutaneous access) and cardiac arrhythmias (via vascular access). Although the MTD technology is similar in the two applications, the constraints are very different. The treatment duration is in the order of 10–15 min for the liver and about l min for the atrium. Hence, while it is acceptable to update the images every 10 s for the liver, such a rate is not sufficient in the heart. On the other hand, the dimensions of thermal lesions induced in the liver are in the order of several centimeters and a few millimeters in the heart. As a result, the spatial image resolution has to be much higher for the imaging of cardiac arrhythmias than for liver tumor treatments. Furthermore, motion (only respiratory versus respiratory and cardiac) that occurs in these two organs also leads to different constraints regarding the MRI acquisition sequences. Therefore, the concept of real-time imaging has to take into account all the hardware and physiological constraints to provide relevant information to the operator. This section focuses on real-time imaging compared to planning and post-interventional imaging that make use of conventional diagnostic imaging techniques. Interventional MRI involves more significant technical constraints than diagnostic imaging because useful information has to be immediately available during the therapeutic intervention. For this purpose, the same image acquisition has to be repeated to analyze and visualize the evolution of contrasts over time in the reconstructed images. Several fast MRI sequences exist, and their choice depends on the requirements in terms of spatial resolution, volume coverage, acquisition rate and radiofrequency energy deposited during the scan in the sample or around an implanted medical device (risk of tissue heating; see the SAR notion in section 2.6.1). A trade-off among acquisition parameters thus has to be met in order to guarantee sufficient signal-to-noise ratio (SNR) while providing suitable contrasts for visualizing the relevant phenomenon. For all such reasons, fast gradient echo sequences, such as FLASH (Markl and Leupold 2012) or True-FISP (Bieri and Scheffler 2013), represent the preferred choice. If the scans have to be accelerated, some variations can be employed, such as echo planar imaging (EPI) (Mansfield 1984) as a multishot EPI (ms-EPI) or single-shot EPI (ss-EPI). These techniques acquire several lines (ms-EPI) or all the lines (ss-EPI) of the k-space following an RF pulse. Therefore, the acquisition time is shorter compared to FLASH, since the number of pulses is reduced to record all the k-space lines, with the highest acceleration factor provided by ss-EPI where all the lines are acquired after a single pulse. However, this choice entails longer echo times, which can decrease the SNR in organs with short T2*. On the other hand, artifacts can occur, including in particular geometric distortions and magnetic susceptibility artifacts around medical devices positioned inside the organs during the treatment. An additional intrinsic constraint in interventional MRI is the reconstruction of images at a rate at least as fast as the acquisition rate. Indeed, if the image reconstruction process leads to delays with respect to the scans, there will be lags between the acquisition and display of useful information for the personnel performing the intervention. Today’s computational performance is capable of providing reconstructed images in real time at rates of at least 10 images/s, which is sufficient for many applications. Among the usually available algorithms on the clinical scanners, parallel imaging techniques (e.g. SENSE, GRAPPA and ASSET) can reduce the number of acquired lines (by a factor of 2 or 3) in the k-space and reconstruct good-quality images in real time by means of simultaneous measurements of an undersampled k-space using multi-element receive coils positioned around the patient. This acceleration can be exploited in several ways, for example, to increase the number of slices for a fixed scan time, reduce the number of lines acquired at each TR, reduce the echo time (decreasing the T2* weighting) or decrease possible susceptibility artifacts or geometric distortions. Another approach consists of simultaneously exciting several slices (simultaneous multi-slices techniques) to gather a composite signal resulting from a mixture of all the slices, and then extracting each slice via a reconstruction algorithm that uses the spatial sensitivities of the different receive coils. This approach can be combined with a FLASH, ms-EPI, or ss-EPI sequence, among others, or include k-space undersampling to benefit from the advantages of parallel imaging and fast sequences. Nevertheless, it should be reminded that the final quality of the images and the eventual artifacts induced by the combination of these acceleration and parallel imaging techniques have to be taken into account. Once the images are reconstructed, they should be processed to extract useful information and deliver it to the operator or MTD to guide the treatment. This typically requires a real-time transfer of reconstructed MRI images to a separate console that performs this operation via specifically developed software for the target application. The provided images can be visualized inside or outside the MRI room depending on the application, for example, when the operator performs the intervention by means of interactive imaging (e.g. insertion of a device into the tumor). The image analysis software should not introduce any latency, especially if high amounts of data are generated at each second. Physiological motion, in particular cardiac and respiratory movements, is quite constraining for MRI. Typically, synchronizing the acquisition of a part of k-space with the respiratory and/or cardiac phase can tackle motion, although at the price of lower temporal resolution, an essential aspect in real-time MRI. On the other hand, using breath holds is difficult to envision. Employing acquisition sequences that are faster than the duration of the considered motion (typically 6 s for the respiratory cycle and 1 s for the cardiac cycle) is thus a viable solution (see section 11.2.1). In this way, the displacement during the acquisition of a given k-space is minimized, although it may be necessary to correct the displacement between subsequent scans for the same k-space plane. The most straightforward way is to use some physiological synchronization (respiratory/cardiac sensor, navigator echo). Another approach consists of correcting the motion that is directly visible on the images of the same slice over time by means of image co-registration. For this purpose, an estimated vector field representing the voxel displacement between an image obtained during the treatment and a reference image has to be calculated. This displacement is then applied to the present image to make it correspond as much as possible to the reference image. The process is then repeated for each newly acquired image. Several algorithms based on rigid and/or deformable transformations have been introduced for different target applications. Such functionalities are particularly important when the analysis of the intensity variation in a pixel during treatment has to be displayed. Another approach to remove motion effects consists of using 3D positioning systems integrated with the MTD (when these are available) and synchronizing the image acquisition with the images of the position of such a system. For example, several existing technologies exploit electromagnetic triangulation systems or MRI measurements from projections along three orthogonal directions by means of small MRI detectors integrated into the MTD. Knowing the instantaneous position in 3D of the organ or medical device also enables the automatic repositioning of a slice at each scan. This dynamic monitoring makes it possible to choose the image refresh rate without necessarily depending on the characteristic time of the physiological motion. However, this requires greater interaction with the MR system by implementing a dynamic feedback loop that analyzes this position in real time to instantaneously reposition the MRI slices over the target region. Interventional MRI involves an MTD and must provide real-time images during an intervention to optimize the use of the device or to guide its operation via the images obtained during the treatment. The variety of possible MTDs includes, for example, single-use consumables, such as a puncture probes, intracardiac catheters or thermoablation systems (radiofrequency needles, microwaves, laser or cryotherapy). These devices are first positioned at the target tissues percutaneously or via vascular pathways (intracardiac catheters) and then used to perform the therapeutic action. Other MTDs are extracorporeal, such as high-intensity focused ultrasound (HIFU), magnetic hyperthermia devices or linear accelerators for radiotherapy. Current clinical MRIs generally consist of a cylinder with an internal diameter of about 70 cm, with 80 cm reached in some new-generation MRI scanners. On the other hand, the length of MRI scanners tends to become shorter, approaching the length of X-ray scanners in which interventional procedures are often performed. However, the available space around a patient remains relatively limited, which makes the positioning of the MTDs via percutaneous access complicated with only MRI guidance. Therefore, the design of MTDs must be adapted to these accessibility constraints. Additionally, the MTD positioning procedure can be performed via alternative imaging modalities, such as ultrasound, by bringing this modality system close to the MRI bed. It should be noted that several MRI manufacturers provide mobile beds, which enable patient preparation and MTD positioning to be done outside the MRI room. Another alternative consists of using interactive imaging for the positioning, but this remains impractical for the operator. MRI compatibility with the MTD is an indispensable prerequisite. A suitable choice of materials composing the MTD can prevent it from being attracted to the MRI magnet. Moreover, the MTD’s magnetic susceptibility should preferably be as close as possible to that of the human tissues to avoid perturbing the spatial homogeneity of the magnetic field B0 in the vicinity of the device. In the negative case, the MRI image will exhibit a loss of signal intensity near the MTD, with the size of the affected area dependent on the employed acquisition sequence (see section 11.2.1), with a more prominent effect in gradient echo sequence, in particular in their accelerated versions such as ms-EPI and ss-EPI. Furthermore, this artifact is more intense when B0 and TE increase and the bandwidth per pixel decreases. Laser fiber and cryotherapy devices have excellent compatibility with MRI, while radiofrequency and microwave systems typically produce stronger artifacts in MRI images. If the MTD emits electromagnetic waves (especially for cardiac ablation radiofrequency catheters, percutaneous radiofrequency needles, or microwaves), it is of critical importance to avoid any electromagnetic interference that could cause artifacts in the MRI images, making them useless. Although these devices operate at frequencies far from those of the clinical MRI systems (around 500 kHz for radiofrequency systems and 1 GHz for microwave systems), the emitted power is in the order of hundreds of watts, which can produce parasitic signals with sufficient intensity at the MRI frequency if the signal emitted by the MTD is not mono-frequential. Since the MRI signal is very low (in the order of microwatts), the parasitic signal produced by the MTD generator and detected by the receiving MRI coils can be larger than the MRI signal. To tackle this problem, a filtering system has to be implemented within the transmitting hardware that links the energy source to the MTD positioned inside the target organ, in particular when the source is located outside the Faraday cage of the MRI scanner. This filtering system can also prevent the transmission of an electrical signal generated by the radiofrequency pulses of the MRI scanner that could be sensed by the electrical cables of the MTD and perturb the operation of the energy source. Several approaches have been introduced to electrically isolate the MTD and the MRI scanner, for example, using passive filters (low-pass or band-stop LC type) or impedance transformers distributed along the transmission line of the MTD. The radiofrequency pulses emitted by the MRI sequence can induce currents in conductive parts of the MTD. A consequence of this is an increased risk of generating hot spots in the tissues in contact with the MTD’s tip. The heating can quickly lead to necroses and irreversible coagulation. The inclusion of stop-band filters tuned to the MRI frequency and distributed along the transmission line at intervals smaller than the wavelength of the MR signal can reduce this effect. Another solution consists of modifying the characteristic impedance of the MTD during the transmission of the radiofrequency pulse by MRI coils (Ozen et al. 2019), which implies an appropriate design of the MTD. Other research groups have proposed using multi-element MRI transmit coils. This approach optimizes the currents in each transmit coil to minimize the currents induced in the MTD (Godinez et al. 2020), and the current in the latter can be measured by an inductive system without requiring specific modifications of the MTD. HIFU and magnetic hyperthermia devices are non-invasive and operate inside the MRI bore. Their considerations regarding access, MRI compatibility and electromagnetic interference risks are analogous to percutaneous MTDs. However, the risks of artifacts in the target region are almost non-existent, which offers more possibilities in the choice of acquisition sequences. The combination of MRI with a radiotherapy device (MR-Linac) is a relatively recent technology. It has the advantage of an MTD located outside the MRI bore, with a modification of the magnet to let the radiotherapy beam pass through it. For the three MTDs mentioned above, one of the challenges is to manage the relative displacement of a moving target (respiratory or cardiac activity) with respect to the local area where the energy is delivered by the MTD. A dynamic feedback system must be devised to control the energy deposition in real time such that this energy is always oriented toward the target and avoids damaging adjacent healthy tissues. This device combines a linear accelerator for radiotherapy with an MRI. It is a completely non-invasive MTD since the radiotherapy emitter is located outside the MRI magnet’s bore. The treatment is performed by radiotherapists and the most used image-guiding modality is X-rays. Radiotherapy treatment is subdivided into various sessions distributed over several days. This means that the target area has to be precisely repositioned with respect to the radiotherapy emitter for each session in order to optimize the delivered dose and avoid damage to adjacent healthy tissues. On the other hand, the geometry of the region to be treated can evolve during the treatment, which ideally requires adaptive approaches. The advantages of combining MRI and linear accelerators are as follows: Today, two clinical systems are available, one employing low-field MRI (0.35 T, MRIdian) and the other using a high magnetic field (1.5 T, Unity Elekta). The low-field system consists of an MRI with two main coils that surround the radiotherapy device. The main acquisition sequences enable the co-registration of the target at each session. During the treatment, cine imaging (True-FISP sequence) can be performed to control the emission/arrest of the radiotherapy beam. The system working at 1.5 T is more recent and includes a Philips scanner that is quite similar to conventional diagnostic scanners, with an aperture for the radiotherapy beam near its center. The advantage of working at 1.5 T lies in images with a higher quality (gain in SNR) or higher spatial resolution and in all the imaging methods available on diagnostic clinical scanners. On the downside, combining high-field MRI with a radiotherapy system involves considerable technological challenges (risk of radiofrequency interference between the waves emitted by the two devices, requiring mutual shielding via dedicated Faraday cages, minimization of radiotherapy overdose effects due to the generation of secondary electrons at some tissue interfaces – the so-called electron return effect). These two systems are used in clinics and aim to provide adaptive radiotherapy for personalized treatment with better control of the radiation dose (higher dose in the target region and lower dose in the organs at risk). Several physical parameters affecting the contrast of MRI images are sensitive to temperature (Quesson et al. 2000). They include longitudinal (T1) and transverse (T2) relaxation times, magnetization (M0) and the resonant frequency of water protons (ν0). Based on the latter, the proton resonance frequency shift (PRFS) technique has become the standard for acquiring images of temperature distribution inside the organs whenever a thermotherapy MTD (radiofrequency, microwaves, laser, HIFU) is used. The variation of ν0 is almost linear over the range of temperatures in liquid water and depends very little on the investigated organ. The variation of ν0 with temperature (the PRF constant called σ in the following) is about 0.01 ppm/°C, which corresponds to a variation of 0.63 Hz/°C for a scanner operating at 1.5 T (ν0 = 63 MHz). Thus, the phase φ of the MRI signal varies as a function of temperature according to the following equation:
11
Interventional MRI
11.1. Introduction to interventional MRI
11.1.1. Intervention planning
11.1.2. Pre-operatory imaging
11.1.3. Post-operative follow-up imaging
X-ray scan
Fluoroscopy
Ultrasound
MRI
Planning
++
–
–
++
MTD positioning
++
++
++
++
Real-time imaging
–
+
Depending on the application
Post-treatment imaging
++
–
+
++
11.2. Technical considerations in interventional MRI
11.2.1. Choice of the MRI acquisition sequence
11.2.2. Image reconstruction
11.2.3. Image analysis and display
11.2.4. Motion management
11.3. Interventional MRI hardware
11.3.1. Intracorporeal medical devices
11.3.1.1. Access and operation of the MTD
11.3.1.2. MRI compatibility and electromagnetic interference
11.3.1.3. Risk of heating in conducting parts of the MTD
11.3.2. Extracorporeal therapeutic medical devices
11.4. MR-Linac
11.5. MRI thermometry for guided thermal therapies
11.5.1. Principle of MRI thermometry
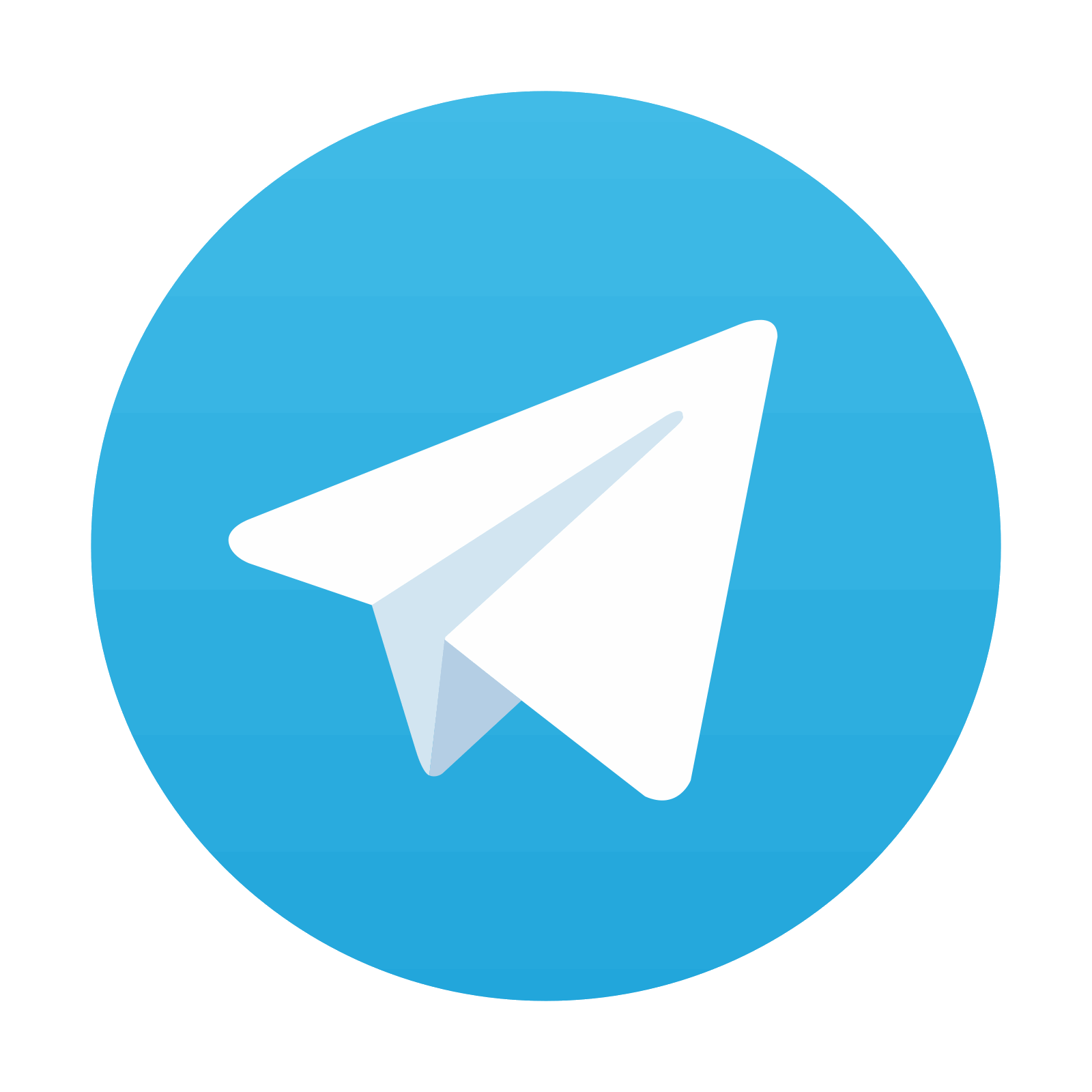
Stay updated, free articles. Join our Telegram channel
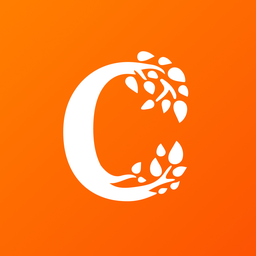
Full access? Get Clinical Tree
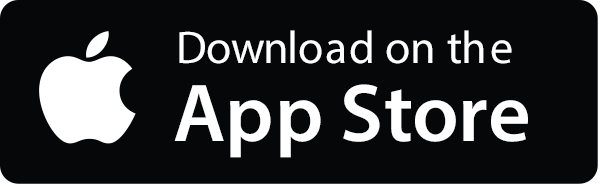
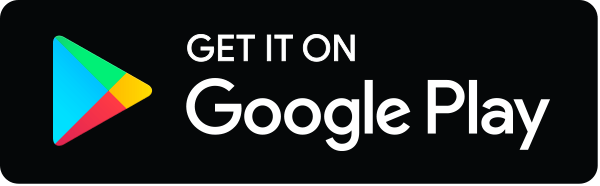