Fig. 1
Characteristic depth-dose distributions of photons, carbon ions, and protons in water
In contrast, protons or heavier particles such as carbon ions enter the body at very high speed. Particles pass through the tissue, slow down, and lose energy in atomic and nuclear interactions in the trajectory in front of the tumor. This reduces the energy of the particles, which in turn causes more interactions with electrons. Maximum interactions occur at the end of range causing maximum energy transfer and thus maximum dose deposition within the target area. The Bragg peak describes the sharp dose increase in a well-defined depth and the rapid dose fall-off beyond the maximum (blue line). Since the width of the Bragg peak is in the millimeter range and usually not wide enough to cover a treatment volume, several of these Bragg peaks must be superimposed to treat a tumor of a certain length and volume, respectively. These superimposed Bragg peaks are called spread-out Bragg peak (SOBP) (Fig. 1, green and yellow line). After the loss of energy at the maximum, the dose drops off abruptly to almost zero. The “residual dose” beyond the Bragg peak is referred to as fragmentation tail. It is formed by interaction of the ions with atomic nuclei in the body, and is usually <10 %. The radiation plan parameters for ion therapy must be very exact as even the slightest inaccuracy in the beam application can lead to imprecision in the range of the ion beam. Optimal treatment plans and precise patient positioning are necessary to obtain a high-dose deposition within the tumor region with maximum protection of healthy tissue surrounding the tumor.
The uncertainty of range of the ions (higher dose concentration at the end of the range of the beam) in human tissue is one of the major hurdles of radiation therapy with ions (Paganetti 2012). For various reasons, the localization of the “actual” dose deposition during treatment can differ from that of the treatment plan, for example, if patient positioning is inaccurate or if the bladder or rectal characteristics change. Therefore, several measurements should be made even during the actual dose delivery, e.g. via positron emitters (PET camera) (Frey et al. 2014) or the induced gamma radiation (Compton camera) (Mackin et al. 2012).
The ratio of Bragg peak dose to the dose in the entrance area is larger for heavy ions compared to protons. Due to their larger mass, angular and energy straggling becomes negligible for heavy ions compared to protons. Therefore, heavy ions have an improved dose conformation as opposed to photons or protons resulting in better preservation of healthy tissue surrounding the target in physical treatment planning. Additionally, heavy ions reveal a strong increase of the LET in the Bragg peak compared to the entrance region (Schulz-Ertner et al. 2006). The biological advantage of high LET radiation was already proposed in neutron therapy, in contrast however, in heavy ion radiotherapy the high LET area can be conformed to the tumor.
2.1 Production of Charged Particles
Compared to photon beams, charged particles have the basic differences that they are slowed down while passing through tissue and thus have a finite range. The energy required to treat a tumor depends on the depth of the target in the body. Treatment with protons requires energy between 80 and 250 MeV. For these energies, cyclotrons are used to produce proton beams with sufficient energies and intensities. The energy required to treat deep-seated tumors with heavy ions is much higher compared to protons. A proton beam of 150 MeV can penetrate 16 cm in water; the same penetration depth for carbon ions can be achieved with energies of 3,000 MeV. To accelerate particles to such high energies, synchrotrons are better suited than cyclotrons. A synchrotron produces pulsed beams, and the energy can be varied from one cycle to the other with a few MeV steps. Thus, modulation of the Bragg peak can be achieved to scan a deep-seated tumor without absorbers, avoiding scattering and degradation of sharpness in energy. However, particle acceleration in synchrotrons is much more difficult and more cost-intensive than in cyclotrons.
2.2 Beam Delivery and Application Systems
2.2.1 Beam Delivery
Most experience with charged particles therapies has mainly been acquired with horizontal beam lines (Fig. 2). The success of a radiotherapy treatment is strongly increased through the possibility of applying the beam to the target using different angles (multiple field irradiation). A gantry for charged particles, however, lies in another dimension compared to photons. Although the weight of a proton gantry is around 100 tons and has a diameter of 10 m, an isocentric gantry for carbon ions has a weight of 600 tons, a length of 20 m, and a diameter of 12 m (Haberer et al. 2004). The enormous size and weight as well as the high spatial accuracy required for the beam position at the isocenter of the target volume was the reason why such a gantry was not built until 2008 in Heidelberg/Germany (Haberer et al. 2004). The first worldwide heavy ion gantry was brought online in October 2012 (Fig. 3).
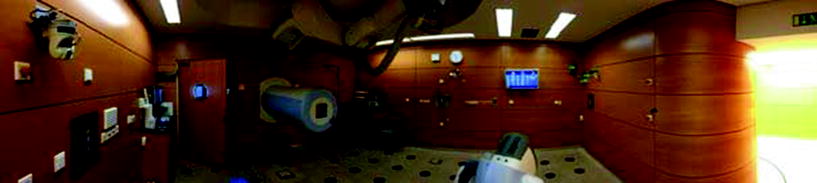
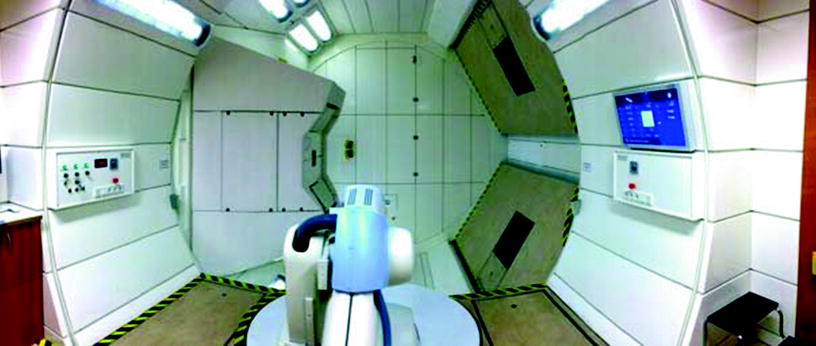
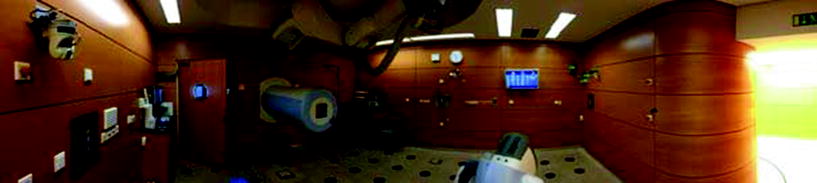
Fig. 2
The interior view of the horizontal beam line treatment room of the Heidelberg Ion Beam Therapy Center (HIT) in Germany (D) in Fig. 4
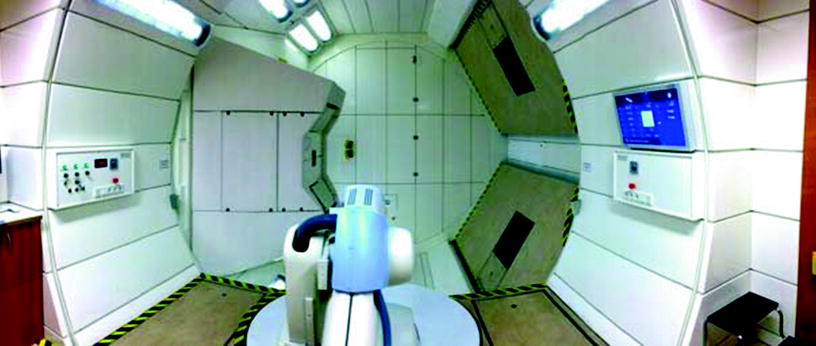
Fig. 3
The interior view of the Gantry treatment room of the Heidelberg Ion Beam Therapy Center (HIT) in Germany, equates (D, F) in Fig. 4
The Heidelberg Ion Therapy Center (HIT) (Fig. 4) provides protons and carbon ion beams using the raster scan technique. Arbitrary particle numbers can be applied to each raster point that is defined by longitudinal range and transverse deflection of a narrow ion beam. This technique of fluence modulation allows generating almost arbitrarily shaped dose distributions in three dimensions, typically only using two beam directions. Treatment plans are usually classified by the way of dose optimization to generate the particle number distributions: single field uniform dose (SFUD: optimization of individual beams yields homogeneous dose distributions for each beam) and intensity modulated particle therapy (IMPT: simultaneous multiple beam dose optimization, may yield inhomogeneous dose distributions of the beams complementing one another). Both algorithms are driven by dose constraints for the target volume and surrounding organs at risk (Schulz-Ertner et al. 2006).
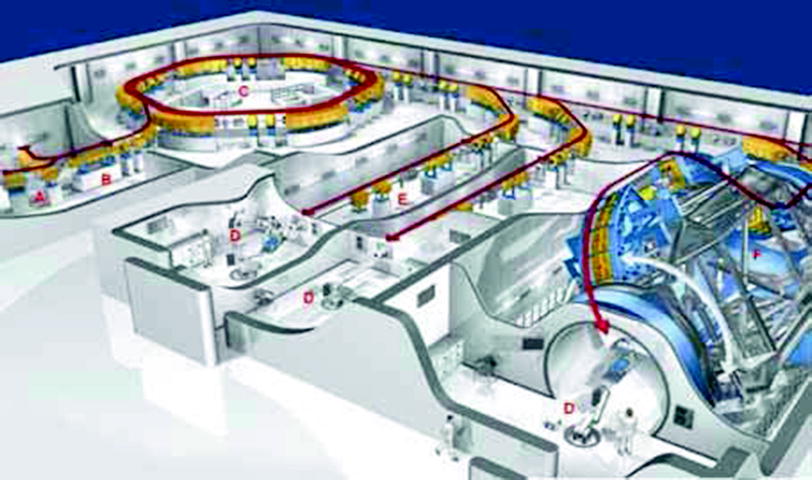
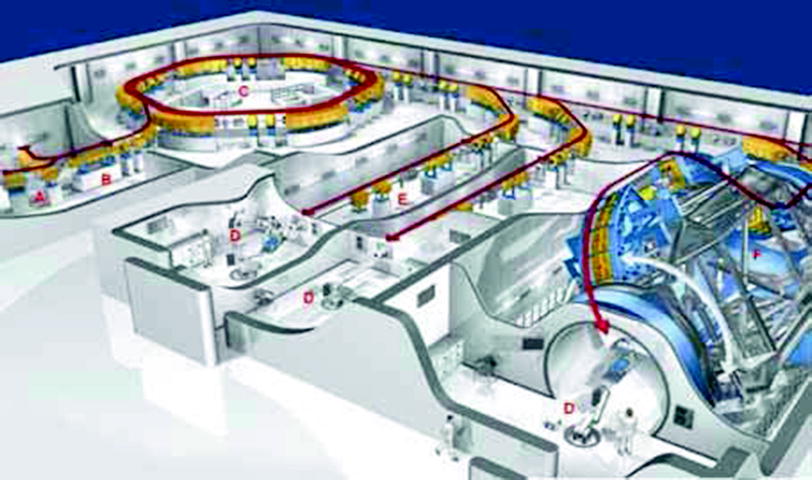
Fig. 4
The build-up of the Heidelberg Ion Beam Therapy center (HIT). In the ion sources (A) ions are produced by snatching parts of the electron cloud of the atoms. The ions are bundled to a beam in electric and magnetic fields and are accelerated to high speed, approximately 10 % of the speed of light, in the linear accelerator (B). In the synchrotron (C), the ions are kept in circular orbits due to superconducting magnets. During approximately one million circulations, the high frequency powered accelerator structures increase the velocity of the ions up to 75 % of the speed of light. On the way to the treatment room (D), the ion beam is conducted and bundled in vacuum tubes (E) by magnets. The world’s first ion gantry (F) with a length of 20 m, a diameter of 12 m, and a total weight of 600 tons, which moves around the patient, went online in October 2012
2.2.2 Beam Application
Two different principle methods for beam application exist, the passive and active beam shaping (Schulz-Ertner et al. 2006). Passive beam shaping was the first method developed and is still the most commonly used method in heavy ion therapy (Fig. 5). After the broadened beam is incoming, the variable range shifter (typically a number of homogeneous plastic plates of different thickness which can be moved into the beam) has to shift the dose into the planned depth. The compensator and collimator are patient-specific devices and adapt the dose distribution to the size and shape of the target volume.
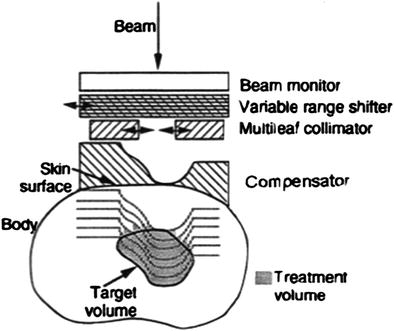
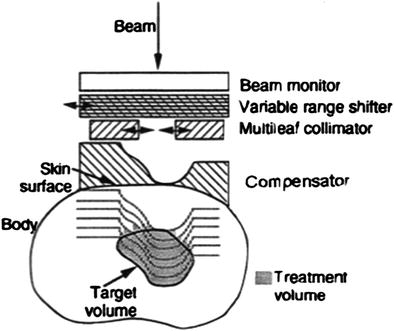
Fig. 5
Principle of passive beam shaping used for charged particle therapy, see text. The lines in the body to the treatment volume represent the distal fall-off that can be shifted to the depth by using a range shifter
In contrast to the passive beam shaping, in which a customized compensator compensates inhomogeneities of the tissue, in the active raster scanning method, the beam intensity and energy range can be modulated by variation. The tumor is divided into isoenergetic layers, which are sequentially scanned (Fig. 6). The advantage of the active raster scan method is the possibility to scan the target volume in three dimensions and the dose distribution can be tailored to any irregular shape without any patient-specific devices, as mentioned above. Additionally, the high-dose region can be conformed to both the proximal and distal ends, in contrast to the passive beam shaping, where only the depth dose can be tailored to the distal end of the target volume (Schulz-Ertner et al. 2006). The integral dose and the target volume receiving high-LET radiation are minimized in case of the active raster scan technique.
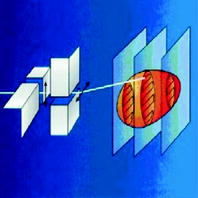
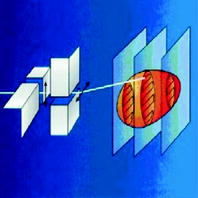
Fig. 6
Principle of active beam shaping. The beam is scanned in vertical and horizontal directions via two pairs of scanner magnets. By switching the energy of the synchrotron, the position of the Bragg peak can be changed resulting in the adaption of the scanned region to the dimension of the target in-depth
3 Biological Principles
The number of ionization events in a certain volume, the so-called ionization density, can specify the effectiveness of a certain kind of radiation. Since this quantity is difficult to measure, linear energy transfer (LET) is mostly used. The LET is a biophysical quantity and describes the number of ionizations per distance and is thus a measure of the effect of ionizing radiation on biological tissue. Sparsely ionizing radiation (low-LET <10 keV/μm) and densely ionizing radiation (high-LET >10 keV/μm) are distinguished. At a cellular level, the high-LET is characterized by a high local dose in the nucleus, thus the possibility of lethal radiation damages increases (Scholz et al. 2001). The higher the LET, the higher the relative biological effectiveness (RBE). The RBE is the ratio of biological effectiveness of one type of ionizing radiation relative to another (here the use of a photon irradiation of a conventional linear accelerator is set to equal one), given the same amount of absorbed energy. RBE can increase with increasing LET to the optimum ionization density; for high LET, however, saturation effects come into effect, which prevent a further increase in action. The RBE of SOBP protons increases with decreasing dose or dose per fraction and increasing depth in the SOBP, with the magnitude of both effects likely being dependent on the α/β ratios of the target cells or tissues (Gerweck and Kozin 1999). The increase in RBE with depth in a SOBP translates into a displacement of the distal edge of the RBE-weighted SOBP to a larger depth (Carabe et al. 2012). Clinical proton beams should always be designed to avoid risk organs at the distal drop-off of the beam. Different tumors respond differently to high-LET radiation. Especially, so-called late-reacting tissues, i.e., prostate tumors, which grow very slowly and usually are not very sensitive to radiation, react to high-LET radiation with increased RBE. The RBE is thus dependent on the tumor entity (nature of the tissue), the single-dose (different local dose distribution), the LET and the ionization density, but independent of oxygenation and cell cycle.
However, the existing experimental biological data is insufficient to define a clear correlation between RBE and dose per fraction or α/β value for in vivo endpoints (Paganetti et al. 2002). In general, it is known that RBE increases with dose-averaged linear energy transfer (LETd), whereas it decreases with increasing dose and α/β value (Gerweck and Kozin 1999; Paganetti et al. 2002; Carabe et al. 2013). An increased RBE due to an increased LETd and dose drop-off could cause a shift in the biologically effective range of the beam by 1–2 mm (Robertson et al. 1975; Paganetti et al. 2000). Several authors contemplated how to allow an estimation of range uncertainties by varying biological effectiveness for treatment planning purposes (Carabe et al. 2012; Bohlen et al. 2012). Biological range uncertainties are an additive to the physical range uncertainties, so treatment sites that involve very inhomogeneous tissues with low α/β values, could benefit from contemplating toward varying RBE values (Carabe et al. 2012).
The RBE value of 1.1 for protons has proven to be a good average representation across the SOBP at any dose and for all tissues (Paganetti et al. 2002), whereas the RBE of carbon ions varies from 1.4 to 5.0 (Jones 2009). If one wants to increase the accuracy of a treatment to levels below 5–10 % of uncertainty, biological effect variations must be considered. Proton RBE is known to be dependent on the α/β value of prostate tissue. The range of α/β values reported for prostate tumors (1.2–5.0) implies that the proton RBE for these tissues could vary significantly compared to the commonly used generic value of 1.1 (Carabe et al. 2013). The uncertainty in the range of the biological dose profile is smaller at higher doses, which could imply that the use of hypofractionated regimens reduces the overall range uncertainty (physical and biological) associated with protons beams (Carabe et al. 2012). Due to these considerations and the fact that proton RBE varies with fractionation, a comparison of proton and photon treatments should be made with caution.
4 Clinical Aspects
The success of irradiation in patients with localized prostate cancer correlates with the administered dose (Hall 2006; Kupelian et al. 2007; Tsuji et al. 2005; Yu et al. 2011; Zelefsky et al. 2001). This is well known for patients with an intermediate risk profile and was also recently found in patients with a low-risk profile (Gleason score <7; PSA <10 ng/ml) (Zietman et al. 2010a). Higher doses lead to higher complication rates particularly to the rectum (bleedings, fistula, and ulcer), urethra (stenosis), and bladder (chronic cystitis).
The rate of side effects is not only dependent on the dose but also on the radiation technique used. In a randomized study in the 1990s, it was noticed that the rectum toxicity was lowered significantly with the application of 3D-CT-based radiation planning compared to simulator-based planning (Dearnaley et al. 1999). In a dose escalation study by the MD Anderson, Pollack et al. described a volume dependency of the rectum toxicity (rectum volume irradiated with >70 Gy: toxicity °II or higher after 5 years 13 % or 51 % by ≤25 % or >25 % rectum volume ≥70 Gy) (Pollack et al. 2002). Due to the use of intensity modulated radiotherapy it is possible to increase the doses up to 76–81 Gy whereat the side effects in comparison to 3D-conformal radiotherapy in the same dosage could be lowered significantly to the level of radiation series with a total dose of 64–70 Gy. This monocentric historic comparison showed a significantly higher cure rate (Zelefsky et al. 2001).
5 Proton Radiotherapy
More than 2,000 prostate cancer patients treated with proton therapy were reported in the literature. Toxicity was in an acceptable range while dose escalation utilizing a proton boost had an improved outcome. Preliminary results with proton only therapy are also available and similar to combined therapy with protons and photons. Dosimetric studies suggest that the greatest benefit for conformal proton therapy lays in a reduced mean integral dose, which could translate into fewer secondary malignancies.
The number of proton beam centers increased rapidly in the last decade, from 20 centers operating worldwide in 2001, to 39 centers in 2011 (available at: http://ptcog.web.psi.ch/ptcenters.html). Proton beam therapy has been used in research applications since the 1950s and entered clinical practice in the first proton therapy center in Loma Linda in 1990 (Jarosek et al. 2011).
To compare the effectiveness of single proton therapy versus photon therapy (IMRT), randomized phase III trials would be needed with extremely long observation times, especially in low-risk prostate cancer patients. Currently, there are no randomized controlled trials and only a few well-conducted cohort studies have compared proton beam irradiation to other treatments.
Commonly mentioned refutations of the detractors of proton therapy on the one hand, say that no evidence exists for the comparative effectiveness or the harmfulness of proton therapy, and on the other hand the high costs of treatment. One report cited that the costs of providing proton therapy were twice as much as any other radiotherapy (Zietman et al. 2010b).
However, the physical dose distribution of protons argues against photons (Fig. 7). It is obvious that parts of the risk organs still remain in the target volume: base of bladder, urethra, and the facing wall of the rectum. The tolerance dose of the urethra, which is at the center of the target volume, is >85 Gy. A prospective study with a fraction scheme of 48 x 1.8 Gy = 86.4 Gy reported urethral strictures of <3 % (Cahlon et al. 2008). For both the bladder and rectum, studies found a high volume effect. The TD 5/5 for radiation of 2/3 of the bladder is 80 Gy, whereas it is 65 Gy for radiation of the whole bladder (Emami et al. 1991). For the rectum, high volume effects are known as well (Kuban et al. 2008). With proton radiation it is possible to especially preserve the posterior rectum wall in comparison to photon radiation, which leads to lesser rectal complication rates. For the proton irradiation we know the retrospective analysis data from Loma Linda (Slater et al. 2004), as well as the randomized study from Boston, in which a proton boost to a total dose of 79.2 Gy versus 70.2 Gy was used after irradiation with photons to a dose of 50.4 Gy. The study showed a significant advantage to the high-dose arm with an acceptable side effect rate (2 % acute and chronic side effects CTC grade 3 or higher) (Zietman et al. 2010a).
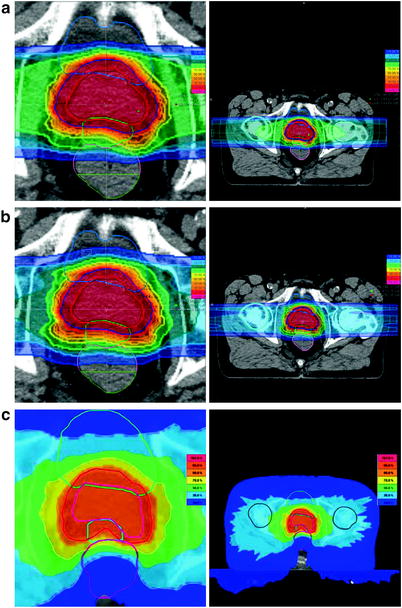
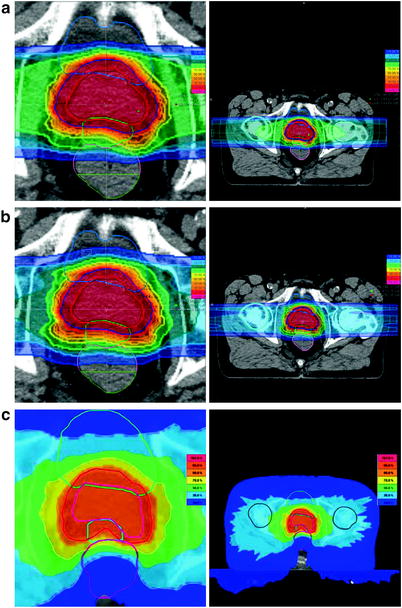
Fig. 7
Comparison of the dose distribution of a carbon ion, b proton, c IMRT (tomotherapy) irradiation of the prostate. To spare the rectum, a spacer gel is placed between the prostate and the rectum (in a + b the green OAR, in c the mint OAR), see Fig. 8. Depicted are the isodoses: dark blue (10 %), light blue (30 %), green (50 %), yellow (70 %), orange (90 %), red (95 %), and pink (107 %) of the applied dose
Concerning the survival rate data, a few major studies were conducted. One of the major dose-escalation studies was carried out at the proton center in Boston. Zietman et al. 2005, 2010a randomized 393 patients with a PSA <5 ng/ml to a low-dose arm (50.4 Gy photon therapy + 19.8 GyE proton boost) and a high-dose arm (50.4 Gy photon + 28.8 GyE proton boost). The analysis revealed a significant difference in biochemical recurrence-free survival in favor of the high-dose arm. Subgroup analysis of low and high risk patients (depending on the Gleason score) showed a significant advantage for the high-dose group in both cases. An impact on the overall survival rate was not observed. Both acute and late toxicities were not increased in either arm compared to the incidence of comparable photon studies. A Japanese phase II study of Nihei et al. 2005 with a similar patient population and treatment approach had similar results and toxicity events.
Boston performed a further dose escalation as well as a comparative study examining photon against proton boost in patients with advanced prostate cancer (T3-4 N0-2) in 202 cases (Shipley et al. 1995). Patients were randomized in a low-dose arm (50.4 Gy photon + 16.8 Gy photons) or a high-dose arm (50.4 Gy photon + 25.2 GyE protons). A statistically significant difference in local control (84 % in the high-dose arm vs. 19 % in the low-dose arm after 8 years) was only observed in the subgroup of less differentiated carcinomas (no effect for the total study population). Regarding late toxicities, the incidence of rectal bleeding was increased significantly in the high-dose arm and the incidence of urethral strictures showed a statistical trend to an increase.
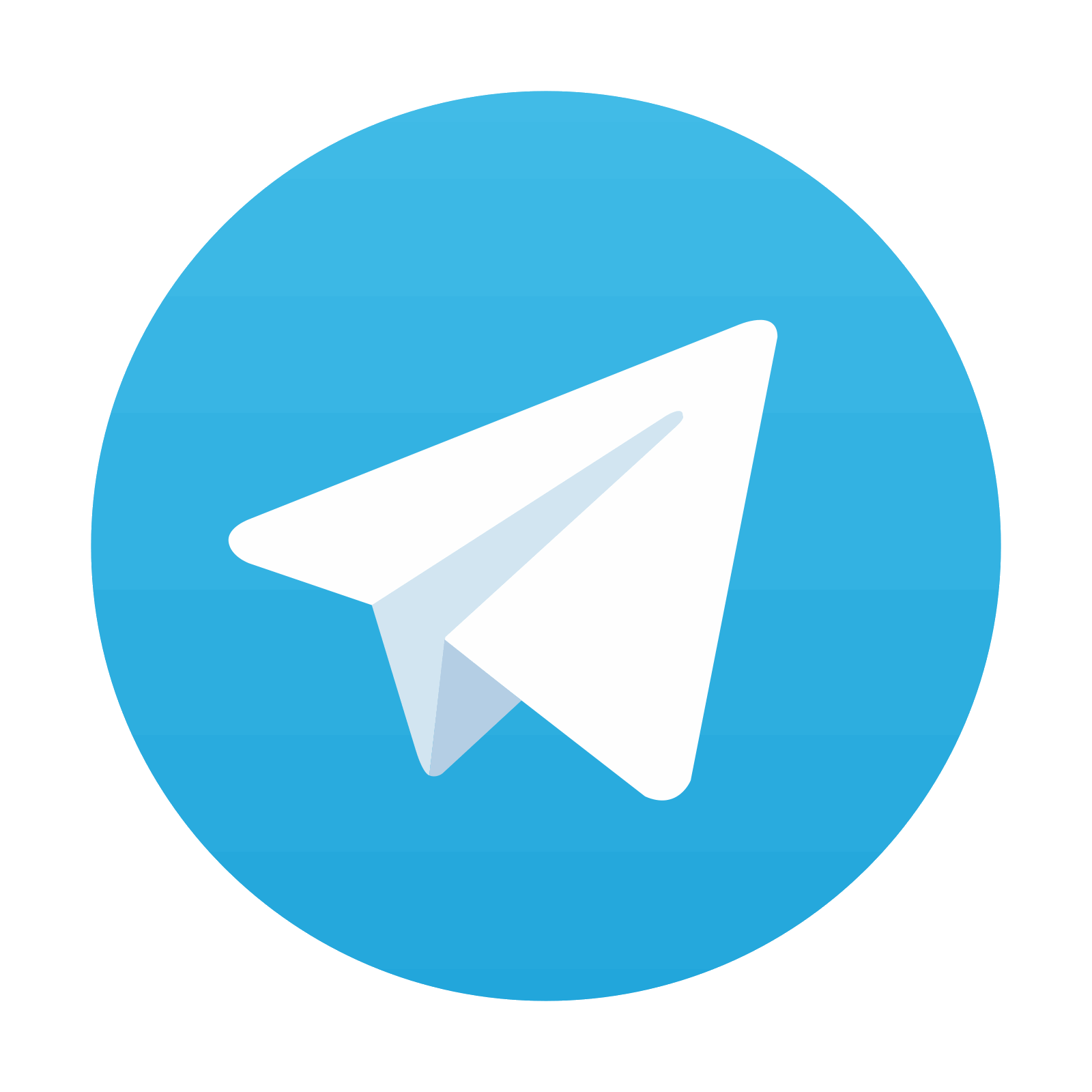
Stay updated, free articles. Join our Telegram channel
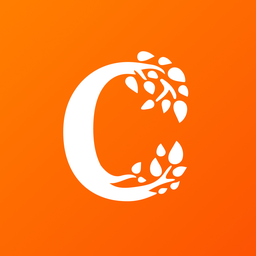
Full access? Get Clinical Tree
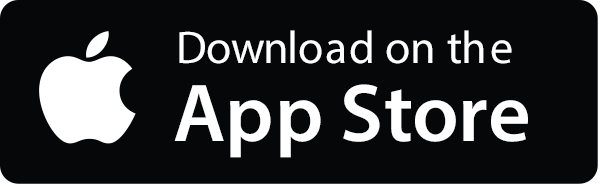
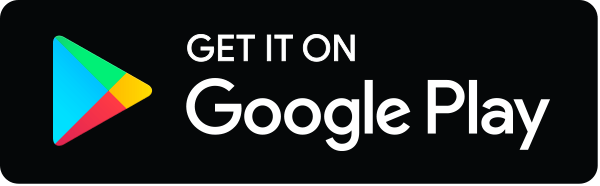