Treatment of glioblastoma multiforme remains a major challenge despite advances in standard therapy, including surgery, radiation, and chemotherapy. The field of nanomedicine is expected to have a major impact on the treatment and management of brain tumors. Over the past decade, significant efforts have been made in using nanoparticles for diagnosis and treatment of brain tumors. One class of nanoparticles, liposomes, have received considerable attention for use as nanocarriers for delivery of therapeutics and contrast agents. The purpose of this article is to present the advances in the design and functional characteristics of liposomes for applications in brain tumor imaging.
Nanomedicine, the application of nanotechnology in the diagnosis and treatment of diseases, is expected to have a major impact on central nervous system–related pathologies. Nanotechnology involves the manipulation and engineering of molecules and molecular constructs at the nanoscale (1–100 nm). Nanomedicine can provide solutions to a variety of problems related to diagnosis and treatment. In the imaging field, the development of nanoparticle contrast agents has allowed for cellular and molecular imaging, monitoring of drug delivery to tumors, and efficient surgical removal of solid tumors. In the therapeutic field, nanoparticles have been shown to increase the bioavailability of poorly water soluble drugs; prevent in vivo degradation of therapeutic molecules, including genetic materials; reduce side effects; and enhance the efficacy of conventional anticancer drugs as well as increase the specificity of delivery of drugs to diseased sites.
Liposomes, one of the most extensively studied nanoparticles, have been investigated for use as a platform technology for the delivery of therapeutic molecules and imaging agents. Liposomal nanocarriers encapsulating cytotoxic molecules, such as doxorubicin, daunorubicin, and cytarabine, have been clinically investigated for treatment of brain tumors. Liposomes have also been tested clinically for delivery of gene therapy products. In preclinical studies using brain tumor models, liposomes have been used for delivery of agents for neutron-capture therapy, increasing the specificity of cytotoxic agents by molecular targeting of tumors cells, as well as convection-enhanced delivery (CED) of drugs.
Liposomes have also received considerable attention for use as a nanoparticle-based MR imaging contrast agent. In comparison to conventional small molecule contrast agents, liposomes provide a unique nanoparticle platform because they confer specific desirable properties with respect to pharmacokinetics, target specificity, therapy monitoring, and signal amplification in contrast agents. MR imaging–based liposomal contrast agents have been preclinically tested and shown to be successful for MR microangiography of the neurovasculature as well as monitoring CED of drugs to brain tumors. This article reports the advances made in the use of liposomes for brain tumor imaging, beginning with a description of various nanoparticle platforms available for use as contrast agents, then describing the design and functional properties of liposomes that make them versatile nanocarriers. Finally, the development of MR imaging–based liposomal contrast agents and their use in brain tumors is described.
Classes of nanoparticles used as contrast agents
Many nanoparticles have been used as contrast agents. The majority of these nanoparticles can be classified within one of the following categories: lipid-based, polymeric, iron oxide, and metal-based nanoparticles.
Lipid-based Nanoparticles
Lipid-based nanoparticles can be subdivided into three categories: liposomes, micelles, and lipid-coated perfluorocarbon (PFC) nanoparticles. A detailed discussion about liposomes is provided later.
Micelles are self-assembled nanoparticles, typically 10 to 50 nm in size, consisting of amphiphilic molecules with a hydrophobic core and a hydrophilic shell. The lipid molecules are typically conjugated to a hydrophilic polymer, such as polyethylene glycol (PEG), to provide long circulation properties to the micellar structures. The hydrophobic core of micelles has been used for delivery of poorly water-soluble therapeutic molecules to solid tumors. Nontargeted and ligand-targeted micelles have been developed for treatment of cancers. For the development of contrast agents, imaging moieties conjugated to amphiphilic molecules have been incorporated into micelles.
PFC nanoparticles are approximately 250 nm in size and consist of a lipid monolayer surrounding a hydrophobic core of liquid PFC. Imaging agents, drug molecules, and targeting ligands can be incorporated into the lipid monolayer. In addition to their role in standard proton-based MR imaging, the ability to encapsulate fluorine atoms within the internal core allows for 19 F-based MR imaging. PFC nanoparticles have been used extensively as molecular imaging contrast agents and multifunctional nanoparticles in cardiovascular and cancer applications.
Polymeric Nanoparticles
Polymeric nanoparticles are fabricated using biodegradable polymers, such as polylactic acid, poly(lactic-co-glycolic acid), polylysine, and PEG-based copolymers. Depending on the type of polymer backbone and fabrication process, polymeric nanoparticles can be prepared in a variety of sizes, ranging from 10 nm to 1000 nm. Dendrimers are highly branched, multigenerational nanoparticles consisting of exterior end groups that can be functionalized with imaging moieties, therapeutic molecules, or specific ligands for tumor targeting. These are spherical in shape and their size increases with the addition of each polymer shell layer. The ability to precisely control the size and functionality of dendrimers makes them one of the most extensively studied nanoparticle platforms. Several dendrimer platforms, including polyamidoamine dendrimers, have been investigated for use as contrast agents and for delivery of therapeutics to brain tumors. In addition to dendrimers, polymeric nanoparticles and micelles prepared using other polymers have been investigated for use as nanotherapeutics and imaging agents in a variety of cancers, including brain tumors.
Iron Oxide Nanoparticles
Iron oxide nanoparticles have been extensively investigated as MR imaging contrast agents for more than two decades. They are the only nanoparticle-based contrast agent approved for use in humans. These nanoparticles consist of an iron oxide core surrounded with an outer coating of hydrophilic polymers, typically dextran, for particle stability. Two types of iron oxide nanoparticles have been developed. Ultrasmall superparamagnetic iron oxide particles (USPIOs) are smaller than 50 nm in size, whereas superparamagnetic iron oxide particles (SPIOs) range between 50 and 200 nm. SPIOs have high T2 relaxivities ( r 2 > 60 mM −1 ·sec −1 ) and are primarily used in T2-weighted imaging. USPIOs have low T2 relaxivities compared with SPIOs and have been investigated for use as blood pool contrast agents. Unlike conventional gadolinium (Gd)-based contrast agents, iron oxide nanoparticles have a blood half-life of more than 18 hours. The presence of dextran on the surface of iron oxide nanoparticles facilitates their uptake by lymphocytes. As a result, these nanoparticles have been investigated in inflammatory-related diseases, lymph-node staging, and cancer imaging. In brain tumors, iron oxide nanoparticles have been used in dynamic contrast-enhanced MR imaging and imaging of the neovasculature as well as evaluating therapeutic response to antiangiogenic therapy. The high-contrast sensitivity of MR imaging combined with efficient cellular uptake of iron oxide nanoparticles has been used for in vivo MR imaging–based tracking of tumor cells, stem cells, and inflammatory cells.
Metal-based Nanoparticles
Two of the most commonly used metal-based nanoparticles are metal nanoshells and quantum dots. Metal nanoshells are composed of a silica core surrounded by a thin metal shell. The most common of these nanoparticles are gold nanoshells consisting of an ultrathin coating of gold. The nanoparticles can be fabricated to absorb or scatter light, depending on the relative dimensions of the core size and shell thickness. Consequently, gold nanoshells have been used as contrast agents in optical imaging. Furthermore, the ability of these nanoparticles to absorb light in the near-infrared (700–1000 nm wavelength) spectrum has facilitated their use in photothermal therapy. Gold nanoshells have also been investigated for thermal ablation of brain tumors in the canine model. Recently, these nanoparticles entered clinical trials for treatment of refractory head and neck cancer. Quantum dots are based on semiconductor compounds consisting of a cadmium-based core surrounded by an inert layer of metallic shell. Similar to gold nanoshells, quantum dots have excellent optical properties that are dependent on particle size. The tunable optical properties of these agents have primarily been used in preclinical optical imaging for a variety of cancer applications, including cellular and molecular imaging of tumors, and in surgical operations.
Liposomes
Liposomes have been used as a platform technology for delivery of drugs and encapsulation of small molecule contrast agents. Liposomes are spherical vesicles made of lipid bilayer, thus resembling tiny cells. The lipid bilayer also serves to separate the internal aqueous core of the liposome from the external medium. Systemically administered liposomes, with diameters ranging between 50 and 400 nm, are much larger than conventional drugs and contrast agents, which are typically subnanometer in size.
Liposomes
Liposomes have been used as a platform technology for delivery of drugs and encapsulation of small molecule contrast agents. Liposomes are spherical vesicles made of lipid bilayer, thus resembling tiny cells. The lipid bilayer also serves to separate the internal aqueous core of the liposome from the external medium. Systemically administered liposomes, with diameters ranging between 50 and 400 nm, are much larger than conventional drugs and contrast agents, which are typically subnanometer in size.
Design and functional properties of liposomes
The size distribution of liposomes depends on their method of fabrication. Two of the most commonly used methods for fabricating liposomes are (1) high-pressure extrusion and (2) sonication.
The high-pressure extrusion process is the most widely used method for fabricating liposomes, with sizes ranging from 50 to 400 nm in diameter. This method demonstrates a high degree of consistency and reproducibility. Liposomes can have a single bilayer (unilamellar) or multiple bilayers (multilamellar), depending on the method of fabrication and particle size ( Fig. 1 ). Liposomes with multiple bilayers are referred to as multilamellar vesicles (MLVs). Unilamellar liposomes, however, are divided into two classes on the basis of size. Liposomes under 100 nm are considered small unilamellar vesicles (SUVs), whereas those larger than 100 nm are considered large unilamellar vesicles (LUVs). MLVs generally have a broad particle size distribution compared with SUVs. The sonication process is primarily used for fabrication of SUVs, typically 30 to 50 nm in diameter.
Liposomes provide several desirable properties in vivo that can be exploited for use in specific imaging applications. For instance, long circulating liposomes have been used as blood-pool contrast agents for MR imaging and CT applications. Ligand-targeted liposomes have been used as molecular imaging contrast agents in MR imaging applications. Multifunctional nanoparticles, developed by coencapsulating an imaging moiety and a therapeutic agent within the same liposome, have been used in image-guided drug delivery. The fabrication of such constructs is facilitated by the characteristics of liposomes, described as follows.
Clearance Route
Small molecule contrast agents are typically cleared via the kidneys. In comparison, liposomes, which are generally larger than 50 nm, have an alternate clearance pathway. Liposomes are primarily cleared by the phagocytic cells of the liver and spleen, referred to as the reticuloendothelial system (RES). Thus, in the context of imaging agents, liposomal contrast agents result in a change in the clearance route from being renal based to RES based.
Pharmacokinetics
The in vivo pharmacokinetics of liposomes is dictated by their surface characteristics ( Fig. 2 ). The clearance of conventional liposomes is initiated by binding of opsonins on the surface of liposomes followed by a cascade of events, which results in their recognition by the RES. Conventional liposomes have a moderate in vivo half life, typically on the order of minutes to few hours. The half-life of liposomes also depends on their size with particles larger than 200 nm demonstrating shorter half-life compared with smaller particles. Their half-life can be prolonged to several hours by decorating the liposomal surface with biocompatible hydrophilic polymers. The most common polymer used in the development of long circulating liposomes is PEG. Pegylated liposomes, also known as stealth liposomes, are able to evade the RES by preventing opsonization, thereby increasing blood half-life to approximately 18 hours. Similar to conventional liposomes, the blood circulation times of pegylated liposomes is also dependent on particle size.
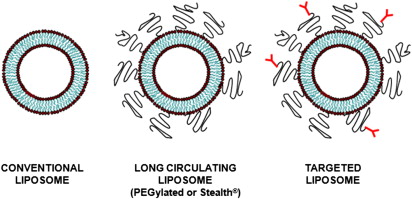
Payload Encapsulation
The central hydrophilic core of a liposome can be used as a nanocarrier to encapsulate a payload, such as a therapeutic or a contrast agent. This construct has been used in several clinically available chemotherapeutic products, such as doxorubicin-encapsulated pegylated liposomes (Doxil), amphotericin-B liposomes (AmBisome), daunorubicin-encapsulated liposomes (DaunoXome), and doxorubicin-encapsulated liposomes (Myocet). In addition to drugs, liposomes have been used to encapsulate iodine and Gd-based small molecule contrast agents. In some cases, the liposomal core has also been used to carry both a therapeutic and a contrast agent, thus allowing for monitoring the delivery of therapeutic agents. This is exemplified by their use in CED of drugs to brain tumors. The hydrophobic bilayer has also been used to carry therapeutic or imaging agents. The encapsulation of chemotherapeutic agents within the liposome offers improved safety and patient tolerance combined with reduced side effects and equivalent or improved therapeutic efficacy.
Targeting to Tumors
Two different mechanisms, passive and active, have been used for targeting liposomes to tumors. Passive targeting takes advantage of the lack continuous lining of endothelial cells and the presence of large pores on luminal surface of tumor blood vessels. Liposomes are able to extravasate through the leaky tumor vasculature and accumulate within the interstitial space of tumor tissue by a process known as the enhanced permeation and retention effect. Their accumulation results in an increase in deposition of chemotherapeutic drug within the tumor tissue, thus improving therapeutic efficacy. The extravasation and accumulation of liposomes within tumors is also size dependent, with larger size liposomes (≥400 nm) showing significantly reduced tumor uptake compared with smaller size liposomes.
Active targeting involves molecular recognition of tumor cells. Ligands ranging from small molecules to monoclonal antibodies have been conjugated to the distal end of PEG chains to facilitate binding of liposomes to cell-surface receptors. Ligand-targeted liposomes delivered via the systemic route can achieve molecular recognition by targeting tumor cells located in the interstitial environment or endothelial cells present on angiogenic blood vessels. Systemically administered liposomes, however, deliver low amount of drug to the tumor cells due to limited transport within the tumor interstitium. Ligand-targeted liposomes have been demonstrated to increase the specificity of delivery of chemotherapeutics by targeting tumor cells that overexpress surface receptors, such as folate receptor, epidermal growth factor receptor (EGFR), and transferrin receptor, among others. In addition to single ligand targeting, liposomes bearing multiple-type ligands that simultaneously target different receptors coexpressed on tumor cells have been investigated.
One final modification that can be used in tumor targeting involves triggered release of liposome-encapsulated contents. The bilayer of liposomes can be modulated depending on the microenvironment to increase the release of the payload. Hyperthermia-mediated delivery of chemotherapeutics from temperature-sensitive liposomal formulation is currently in clinical trials for the treatment of patients with locally recurrent breast cancer.
MR imaging–based liposomal contrast agents
For more than two decades, liposomes have been used as MR imaging–based nanoparticle contrast agents. The usefulness of liposomal contrast agents has been demonstrated in small animal models. For the development of T1-based liposomal contrast agents, three different constructs have been used: (1) core-encapsulated Gd (CE-Gd) liposomes, (2) surface-conjugated Gd (SC-Gd) liposomes, and (3) dual-Gd liposomes ( Fig. 3 ). In CE-Gd liposomes, small molecule conventional Gd chelates are encapsulated within the core of the liposome. In SC-Gd liposomes, the Gd chelates are conjugated on the surface of the lipid bilayer. Dual-Gd liposomes comprise two pools of Gd: core encapsulated and surface conjugated. For MR angiography applications, long circulating liposomal-Gd contrast agents provide uniform signal intensity and stable enhancement over an extended period of imaging.
The T1 relaxivity of liposomal-Gd contrast agents is determined by interactions between the Gd atoms and the bulk water molecules. The encapsulation of Gd chelates within the interior core of the liposomes results in lower T1 relaxivities for CE-Gd compared with conventional contrast agents. The low T1 relaxivity of CE-Gd is a result of slow transport of water molecules across the lipid bilayer, rendering external bulk water relatively inaccessible to the relaxing influence of the encapsulated Gd atom. The T1 relaxivity of CE-Gd agents can, however, be increased by decreasing particle size. In spite of reduced T1 relaxivity, the advantages of CE-Gd liposomes have been demonstrated in preclinical imaging studies. In a rat model, conventional contrast agents demonstrated rapid extravasation from normal vasculature into the surrounding extravascular space. Consequently, higher background signal and low vessel conspicuity were obtained, limiting visualization of the spinal microvasculature. Alternatively, CE-Gd liposomes demonstrated low background signal and higher vessel conspicuity because of negligible extravasation from the vascular compartment. As a result, CE-Gd agent demonstrated visualization of spinal vasculature, including the artery of Adamkiewicz and the venous plexus. The superior imaging characteristics of CE-Gd agents have also enabled excellent visualization of the mouse cardiovascular structures, including the coronary arteries. The uniform and steady signal enhancement provided by long circulating CE-Gd agent enabled accurate and repeated quantitative assessment of cardiac function for mouse phenotyping.
SC-Gd agents represent a second-generation liposomal-based MR contrast nanoparticle. Compared with CE-Gd agents, SC-Gd agents show higher T1 relaxivities because the lanthanide center is readily accessible to bulk water. In a mouse model, the higher T1 relaxivity of SC-Gd enabled ultrahigh-resolution imaging of neurovasculature at approximately 50 μm in-plane resolution. Excellent visualization of the circle of Willis and perforating vessels was demonstrated using SC-Gd ( Fig. 4 ). In addition to visualizing the arterial blood supply, SC-Gd agents enabled simultaneous visualization of the venous system.
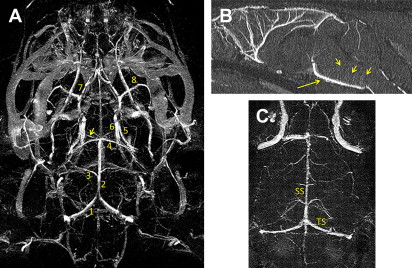
More recently, a third-generation liposomal-Gd agent, referred to as dual-Gd, was developed. Dual-Gd agents demonstrate high nanoparticle-based T1 relaxivity compared with previous generations of liposomal contrast agents. The development of ligand-bearing dual-Gd constructs could, therefore, serve as a signal amplification vehicle for molecular imaging because they can deliver several thousand Gd moieties to each cell-surface receptor of the targeted cell.
Liposomal nanoparticles have also been investigated for their use in T2-weighted imaging. These agents typically consist of USPIOs that are encapsulated within the core of the liposome. The sequestration of USPIOs within the liposome interior results in a high T2 relaxivity compared with the free USPIOs.
Liposomes in brain tumor imaging
Although systemically administered liposomes and other nanoparticles can extravasate and accumulate in tumors through compromised vasculature, their migration within the tumor interstitial space is slow and limited. Small molecules, such as conventional therapeutic and contrast agents, rapidly diffuse in and out of the tumor via brownian motion. Alternatively, macromolecular constructs, such as nanoparticle contrast agents, are diffusion limited, moving slowly or not at all within the tumor interstitium. One approach to overcoming this limitation is the use of CED, a drug delivery system designed at the National Institutes of Health in 1994. In CED, a canula is surgically placed into the targeted lesion via a burr hole and the drug or contrast agent is actively infused. Positive pressure gradients resulting from direct intracerebral infusion cause bulk flow and result in distribution of the agent throughout the target volume. Thus, unlike intra-arterial infusion, CED is not limited by diffusivity within the tumor bed. During the initial infusion phase, positive pressure is used to distribute the infusate throughout the prescribed volume. Once pressure equilibrium is achieved, diffusion rather than bulk flow of the agent into the surrounding perimeter occurs. Furthermore, the ability of therapeutic or contrast agents to bypass the blood-brain barrier facilitated by CED allows for distribution beyond the tumor margins, where an intact blood-brain barrier would be expected to limit deposition via vascular infusion.
CED, similar to other techniques, demonstrates some limitations. Firstly, it is invasive, requiring surgical placement of a canula and complicated infusion techniques. Secondly, although canula placement can be tailored to distribute asymmetrically, distribution for the most part is spherical around the point of infusion.
Although it may be possible to overcome this second limitation by infusing a larger amount and distributing the agent over a larger tissue volume beyond the tumor margin, this can result in a third limitation, overtreatment. Unlike other ablative technologies, however, in which there is a sharp zone of transition between the treated and nontreated tissue, CED has a zone of transition in which diffusion peripheral to the actively infused agent occurs, thus potentially treating those tumor cells that have infiltrated beyond the zone.
A technical limitation caused by the canula design, which resulted in backflow of the infusate, has been addressed with the development of reflux-free stepped canulas, resulting in a more accurate distribution of nanoparticles.
The coupling of liposomal-encapsulated chemotherapeutic drugs with CED for treatment of brain tumors has been extensively investigated in preclinical models, confirming safety and feasibility. A major limitation with CED of liposomal therapeutics, however, has been the difficultly in monitoring the delivery and distribution within brain tumors. To circumvent this problem, Saito and colleagues pioneered the use of Gd-encapsulated liposomal agents for MR imaging–based real-time monitoring of convection-enhanced delivery (CED) of therapeutics into brain tumors. Preclinical CED studies conducted in rodents and primates demonstrated that Gd-encapsulated liposomal contrast agents not only predict delivery but also allow for an accurate correlation between the infused and final volume of distribution. To further optimize the CED method for delivery of liposomal therapeutics, studies have been conducted on liposomes coencapsulating both Gd contrast agents and chemotherapeutic molecules. The same group has recently published their preliminary results on CED of coencapsulated liposomal agents in a primate brain. These potentially mark the final phase of preclinical trials before translating into clinical trials.
Summary
The versatile nature of liposomal platforms has proved its usefulness as a multifunctional nanoparticle for brain tumor imaging. The ability to coencapsulate therapeutic molecules and imaging agents within liposomes coupled with surface decoration allows for the targeting of cell-surface receptors overexpressed on brain tumor cells, such as EGFR or EGFRvIII. The latter has the potential to expand the usefulness of CED-based approach in the treatment of brain tumors. In addition to CED-based methods, liposomal-based multifunctional nanoparticles could be delivered systemically for monitoring the efficacy of neoadjuvant brain tumor therapies. The long circulating property of liposomal contrast agents could also be used for measuring nanoparticle-based transvascular permeability in brain tumors as well as accurate fractional blood volume measurements during antiangiogenic therapy. Given the encouraging results from current preclinical studies, it seems that human clinical trials would be expected. Moreover, liposomal applications in brain tumor imaging might surface as an adjuvant modality for diagnosis and targeting of tumors as well as monitoring therapy response.
Disclosures: Dr Ghaghada and Dr Mukundan are stockholders in Marval Biosciences Inc, a start-up company developing nanoparticle contrast agents for use in x-ray and CT imaging.
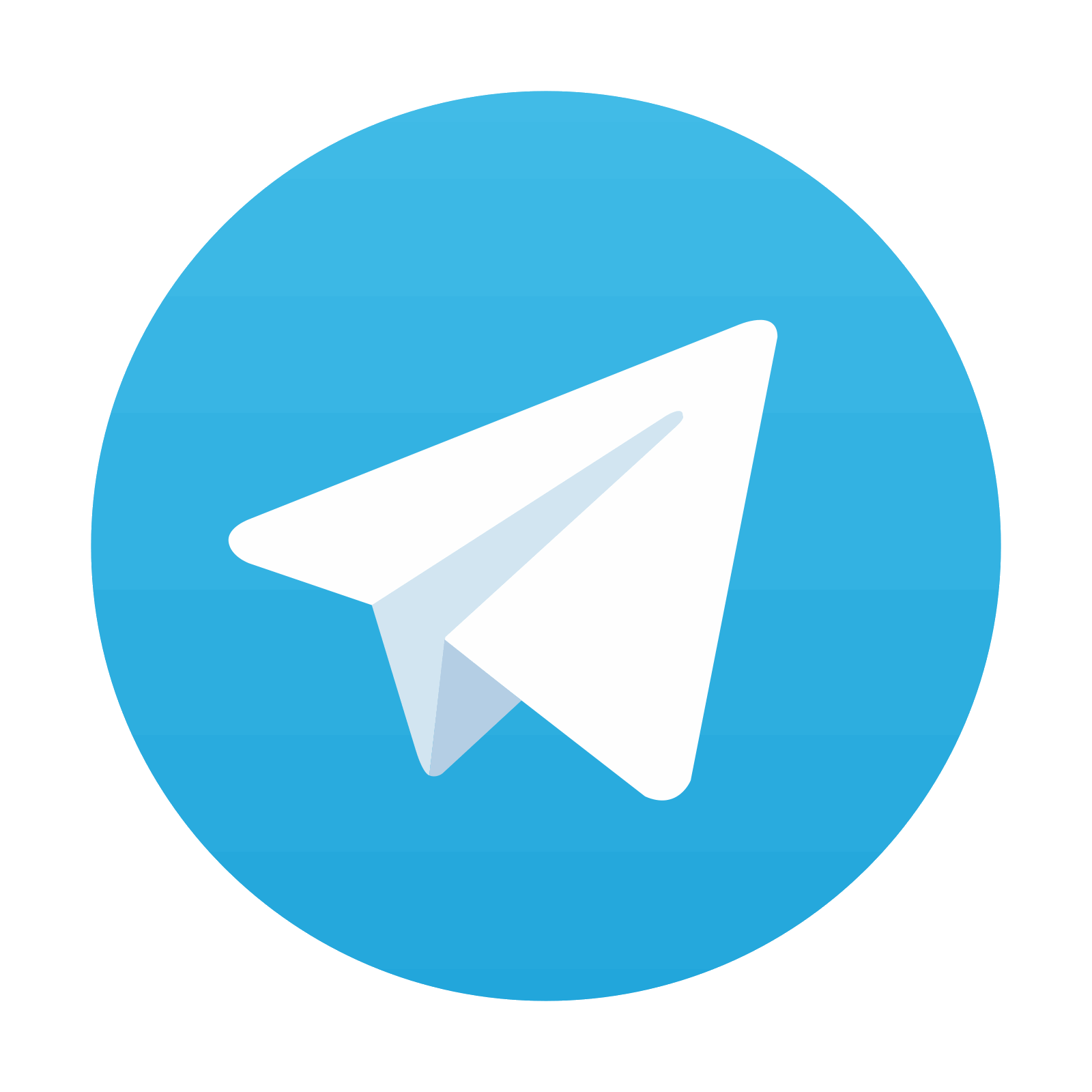
Stay updated, free articles. Join our Telegram channel
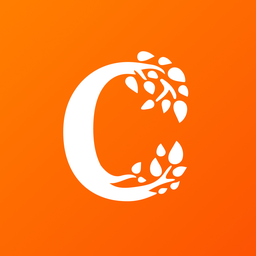
Full access? Get Clinical Tree
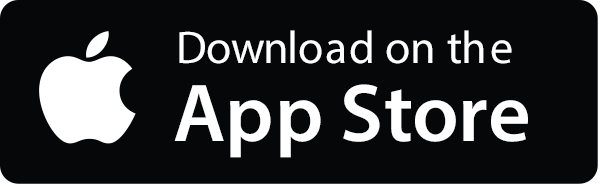
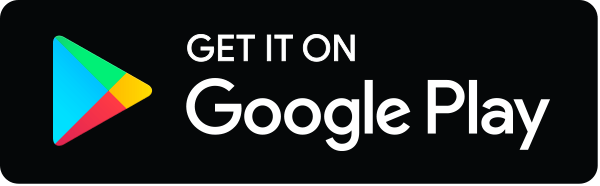