227CHAPTER 15
Liver SBRT
Radiation therapy (RT) continues to grow as an important modality for the treatment of liver lesions. Historically, RT has not been used as a definitive therapy because of concern of liver tolerance, limited technology to deliver ablative doses of radiation, lack of clinical data, and availability of other liver-directed therapies. Advancements in the past two decades have led to expansion of RT for liver tumors in many forms including conventional fractionated external beam radiation therapy (EBRT), stereotactic body radiation therapy (SBRT), also known as stereotactic ablative radiation therapy (SABR), charged particle therapy, brachytherapy, and transarterial radioembolization techniques. Accumulated knowledge in the setting of conventionally fractionated EBRT has established that higher doses lead to better local control (1–3), paving the way for the emergence of liver SBRT. The purpose of this chapter is to discuss the development of SBRT and its role in the management of liver tumors. We review patient selection, simulation, treatment delivery, posttreatment surveillance, and treatment outcomes. An important point worth noting, however, is that although SBRT in the United States is restricted to ablative doses of radiation delivered in five fractions or less to comply with medical billing reimbursement codes, hypofractionated courses of RT using greater than five fractions have achieved similarly high rates of local control (4). For the purposes of this chapter, the term “SBRT” refers to any hypofractionated radiation schedule that can achieve tumor ablation.
HISTORY OF LIVER-DIRECTED RADIATION THERAPY
EBRT was restricted for palliative intent treatments in the past primarily because of concerns of radiation-associated liver disease (RILD), a phenomenon first described in 1965 in a cohort of 13 patients who underwent whole-liver irradiation (5). The histopathological correlation is known as veno-occlusive disease (VOD), manifesting micro-scopically as occlusion of the central veins, sinusoidal congestion, and centrilobular hepatocyte loss (6). Clinically, the classic signs of RILD include an acute triad of hepatomegaly, ascites, and elevation in alkaline phosphatase (ALP) followed by the development of anicteric ascites approximately 2 weeks to 4 months after hepatic irradiation (7). Although the majority of patients recover completely in 3 to 5 months, a minority of patients will experience progressive liver fibrosis and failure, with the possibility of fulminant liver failure (8, 9).
Subsequent studies have demonstrated a volume effect on the likelihood of developing RILD. Recognizing that partial liver volumes could tolerate much higher doses of radiation, investigators described the risk of developing RILD using a normal tissue complication probability (NTCP) model as a function of mean dose to the liver (7, 10). As technological advances paved the way for delivery of more focused and precise RT, specifically with advances in imaging techniques, the advent of three-dimensional radiation planning, respiratory motion management, and image-guided RT, there 228has been renewed interest in modern liver-directed EBRT with results demonstrating potential for improved outcomes through further intensification of local therapy.
Because of the promising results of fractionated conformal EBRT, investigators pursued a strategy of further dose escalation that was enabled by the development of SBRT. SBRT for the treatment of intrahepatic malignancies was first reported in 1995 by Blomgren et al. at the Karolinska Institute in Stockholm (11), in which the stereotactic radiosurgery (SRS) experience for treating brain tumors was adapted to treat extracranial targets in the liver, lung, and retroperitoneum.
Why SBRT?
SBRT allows for the delivery of potentially ablative doses of radiation with rapid dose falloff at the periphery of the target. Through precisely targeting tumors and minimizing dose to normal liver, SBRT has shown excellent local control rates without higher rates of RILD (4, 12–24). There has since been accumulating clinical experience with SBRT for definitive management of primary lung (25, 26), pancreatic (27, 28), liver (16, 29), bile duct (30), kidney (31), and prostate cancers (32, 33), as well as for aggressive local treatment of metastatic tumors to the liver (34) and lung (35).
INDICATIONS
SBRT to the liver is indicated for a variety of clinical conditions. The most common primary liver tumor is hepatocellular carcinoma (HCC) (36). Patients treated with SBRT for HCC often have an underlying background of cirrhosis, which can be an independent cause of major morbidity and mortality. These patients may already have compromised liver function prior to any therapy. Historically, surgical resection has been the primary curative modality for patients with HCC; however, most patients are ineligible for surgery because of extent of disease or concern for hepatic decompensation, especially in cases with advanced cirrhosis. Alternative treatments include liver transplantation, radiofrequency ablation (RFA), microwave ablation, transarterial radioembolization, and transarterial chemoembolization (TACE), the individual details of which are outside of the scope of this chapter. SBRT represents a noninvasive locally ablative treatment for localized HCC unsuitable for surgery. In addition, because up to 30% of patients with HCC awaiting liver transplantation may be removed from the transplant list because of progressive disease (37), SBRT can also serve as a bridge to liver transplantation for patients who are not amenable to other liver-directed therapies (38).
After HCC, intrahepatic cholangiocarcinoma (ICC) represents the second most common type of primary liver tumor (39). The treatment paradigm for ICC is similar to that of HCC, as surgery is considered to be the primary curative modality. Unfortunately, many patients are either medically unfit for surgery or present with unresectable or metastatic disease, and the prognosis for unresectable ICC is dismal, with less than 5% of patients surviving over 5 years (40). Clinical studies in the setting of conventional EBRT (41–43) have demonstrated a clinical dose–response relationship, which has motivated investigations into dose escalation to improve disease control and survival. SBRT represents the current frontier of dose-escalated RT for unresectable ICC.
The liver is a common site of metastatic spread for many tumors in the gastrointestinal system, particularly colorectal cancer (CRC). Standard treatment for metastatic CRC involving the liver is surgical resection for patients with oligometastases limited to the liver, along with combination systemic chemotherapy (44). Unfortunately, only approximately 20% of patients present with resectable hepatic metastases (45), and the remainder of patients are unresectable because of the presence of diffuse burden of disease in the liver, unfavorable anatomic tumor location, and/or medical comorbidities (46). SBRT will likely play a larger role in patients with oligometastatic disease given improvements in various systemic therapies for a variety of histologies that justify locally aggressive treatment. It may also be combined with other established liver-directed therapies to optimize control and toxicity.
SRS is a well-established treatment for intracranial arteriovenous malformations (AVMs) (47), and there is emerging evidence of its utility for spinal AVMs (48, 49). However, there is limited evidence on the use of RT for management of hepatic AVMs. Hsu et al. reported on the efficacy of 3D-conformal RT for arteriovenous shunting in patients with HCC (50). The investigators concluded that RT may be an appropriate and safe option to obliterate the shunt and may also allow patients with underlying HCC to receive future chemoembolization, which is traditionally contraindicated in the setting of an arteriovenous shunt. To our knowledge, there are no reports in the literature on SBRT for hepatic AVMs.
229Preclinical data have suggested that RT may act synergistically with immunotherapy to stimulate antitumor immune responses (51). The optimal dose and fractionation for triggering immune responses remain unclear (52), but there is excitement about combining SBRT and immunotherapy in many tumor types (53). Given that HCC is an inflammation-induced cancer associated with hepatitis B and C infection and other causes of chronic inflammation, immunotherapies may be particularly effective in treating HCC alone or in combination with SBRT (54). For example, a preclinical model of HCC in mice has suggested that concurrent treatment with an anti-PD-1 antibody can augment the effect of SBRT (55). MD Anderson Cancer Center is currently enrolling patients on a phase I/phase II clinical trial combining tremelimumab, a monoclonal antibody against cytotoxic T-lymphocyte-associated protein 4 (CTLA-4), with TACE, RFA, and SBRT for patients with HCC or biliary tract carcinomas (NCT01853618), and multiple clinical trials are investigating immunotherapy as a monotherapy for HCC.
In the setting of liver metastasis, combining SBRT with immunotherapy is particularly attractive. There have been several clinical case reports of patients treated with RT in combination with immune checkpoint inhibitors leading to an abscopal effect, or tumor regression outside of the radiation field (56–58). Many tumors with propensity to metastasize to the liver, including lung cancer (59) and a subset of colon cancers (60), have shown excellent responses to immunotherapies in clinical trials. Thus, combining liver SBRT with immunotherapy in hopes of triggering systemic cancer immune responses represents a promising clinical approach for metastatic tumors that is under investigation in several clinical trials.
PATIENT SELECTION
Patient selection is a key component of the decision process when choosing among the many available local treatment options. In general, multidisciplinary tumor board discussion is recommended to determine the optimal treatment for each individual patient (61). The ideal SBRT candidates are patients with small, peripheral tumors located away from the bowel, chest wall, or central liver. These patients should have a limited number of lesions (one to three), and have underlying Child–Pugh (CP) class A (CP A) liver function (Table 15.1). In practice, because other established liver-directed therapies including surgical resection play important roles in treating liver tumors, many patients may not present with ideal lesions. As such, it is important to involve a multidisciplinary management team to individualize the best treatment approach for each case.
It is also important to know the limitations of other therapies. For instance, RFA is not appropriate 230for tumors next to large vessels because of the heat-sink effect, near bowel because of concern of bowel damage, or near the diaphragm because of difficulty in accessing the tumor (62, 63). In addition, local control for tumors greater than 2 cm with RFA is significantly worse than for tumors less than 2 cm (64–68). TACE is not suitable for tumors with portal venous tumor thrombus because of ischemic concerns, and it is not considered to be ablative, with recent data showing that local control with TACE is inferior to that with SBRT (69). Liver transplant for HCC is limited by the supply of liver donors, and surgical resection is limited by the ability to achieve a negative margin and the ability to leave a viable liver remnant.
TABLE 15.1 Patient Selection Factors for Liver SBRT
Selection Factor | Ideal Parameters for Liver SBRT | Exclusion Criteria Parameters |
Patient immobilization | Able to tolerate immobilization | Unable to tolerate immobilization |
Imaging | Tumor clearly defined on triphasic enhanced CT or MRI (HCC) or contrast-enhanced CT or MRI or PET (metastases or CC) |
|
Eligibility for other therapies | Ineligible for resection or other local therapies because of technical considerations or concerns of efficacy and/or toxicity |
|
Liver function | Child–Pugh class A | Child–Pugh class C |
Healthy liver volume | Ability to meet dose constraints | <700 cm3 remaining healthy liver volume |
Tumor location | >1 cm from critical OARs, such as bowel, diaphragm, chest wall, or central liver | <5 mm from critical OARs |
Great vessel involvement | May be involved |
|
Number of lesions | 1–3 lesions | Five or more intrahepatic lesions |
Burden of extrahepatic disease | None | Uncontrolled or significant extrahepatic burden |
CC, cholangiocarcinoma; HCC, hepatocellular carcinoma; OAR, organ at risk; SBRT, stereotactic body radiation therapy.
More importantly, however, is a better understanding of organ tolerance and how to safely deliver ablative doses to determine the role of SBRT, particularly for tumors that are not suitable for surgical and interventional approaches. Planned optimization with inverse planning allows the ability to conform the dose away from critical adjacent structures to reduce toxicity. In addition, because the prescription dose and target coverage can be adjusted to meet dose constraints, the treatment is not given in the same “all-or-none” manner that limits other local therapies, thus further expanding the range of clinical scenarios for which SBRT may be used. Therefore, a universal maximum with regards to tumor size or number of targets does not exist. SBRT is limited only by the ability to meet tissue constraints, which is based primarily on a combination of tumor size, number of lesions, location, amount of normal liver parenchyma, and underlying liver function.
Exclusion criteria vary between protocols, but, in general, patients with multiple (≥5) intrahepatic or significant uncontrolled extrahepatic burden of disease, poor underlying liver function (CP class C) or healthy liver volume (<700 mL), very large tumors (>6 cm), and tumors in close proximity (<5 mm) to organs at risk (OARs) are poor candidates for SBRT (Table 15.1). It should be noted that age and histology of disease do not serve as inclusion or exclusion criteria. To optimize the benefit of SBRT, ideal patients should have limited active extrahepatic disease.
IMAGING
High-quality imaging to delineate target lesions is a crucial first step in safely implementing SBRT. For HCC, arterial enhancement with delayed washout is the hallmark radiographic appearance. Therefore, patients should undergo a triphasic contrast-enhanced (hepatic arterial, portal venous, and delayed phases) CT scan or multiphase dynamic MRI of the liver (Figures 15.1 and 15.2). HCC will appear hyperintense on the arterial phase while hypodense on the portal venous and delayed phases, as contrast “washes out” from the tumor (70). Triphasic contrast-enhanced CT scans are conducted with 1.25-mm slice thickness, as the thinner slice thickness provides greater volumetric resolution of the anatomy. In addition, MRI may provide improved intra-hepatic soft tissue resolution (Figure 15.2F), and this complementary information may be especially useful for target delineation, especially with tumors that are difficult to visualize on CT, such as ICC. 18F-fluorodeoxyglucose (18F-FDG) PET may provide additional information in localizing the existing metabolically active tumor as well as detecting occult or new tumors that have developed in the interim between diagnostic imaging and treatment planning (71, 72).
FIGURE 15.1 Diagnostic triphasic contrast-enhanced liver CT of a patient with hepatocellular carcinoma. (A) Tumor enhances on arterial phase because of influx of contrast secondary to tumor hypervascularity (white arrow), with subsequent hypodense appearance because of rapid washout of contrast from tumor on portal venous phase (B), and delayed phase (C). There is presence of portal vein invasion (black arrow).
231
FIGURE 15.2 Target delineation using multiple imaging modalities. (A–C) Serial axial CT images showing contoured target volumes and associated organs at risk. Diagnostic imaging that was fused to the treatment planning scan and used for target delineation includes (D) arterial phase and (E) portal venous phase of triphasic contrast-enhanced CT, as well as (F) MRI for improved soft tissue resolution. GTV, cyan;PTV, red; chest wall, magenta; healthy liver volume, green; portal vein, blue; central hepatobiliary tract, brown; spinal cord, yellow.
GTV, gross tumor volume; PTV, planning target volume.
SIMULATION, MOTION MANAGEMENT, AND TARGET DELINEATION
Hepatocellular Carcinoma
Patients are generally simulated in the head-first-supine position with arms extended above their heads. At our institution, fiducial markers are typically implanted prior to simulation, unless other landmarks or surrogates can be used for tumor tracking, such as lipiodol from prior TACE procedures, indwelling catheters or stents, or the diaphragm for tumors near the dome of the liver. Multiple types of fiducials exist, with selection influenced by needle caliber and length, as well as anatomy and characteristics of target lesion (73, 74). Marsico et al. assessed the therapeutic usability of gold-notched flexible anchor wire (0.28 mm × 10 mm [contained in 25-gauge needle]) and gold cylindrical grain (1 mm × 4 mm [contained in 17-gauge needle]) in radiosurgery for liver tumors, and determined that the grain fiducial markers resulted in better CT visualization and less incidence of minor late complications compared with the anchor markers (75).
As stated earlier, arterial imaging is important; thus, patients undergo both an early arterial-phase CT scan acquired at end-expiration breath-hold and a venous-phase CT scan also acquired at end expiration. Slice thickness is generally 1.25 mm. Following these scans, a four-dimensional (4D) CT scan is obtained to capture respiratory motion as well as to serve as a delayed-phase scan.
Delineation of the gross tumor volume (GTV) should be done with the aid of any and all diagnostic scans including CT and MRI. The target volume includes the radiographically apparent arterially enhancing and/or washed-out tumor seen on the arterial and venous phases, respectively, including any arterially enhancing vascular tumor thrombus. Bland vascular thrombi will show no change in enhancement pattern between phases (76) and should be excluded. Additional margin for clinical target volume (CTV) to encompass microscopic tumor extension is not routinely done. The Radiation Therapy Oncology Group (RTOG) has published consensus guidelines on HCC contouring (77). OARs include normal liver parenchyma, spinal cord, heart, chest wall, esophagus, kidneys, stomach, small and large bowel, and central hepatobiliary tract (cHBT), defined by a 15-mm expansion of the portal vein from the splenic confluence to the first bifurcation of left and right portal veins (78, 79). Guidelines for dose constraints to these OARs are reviewed in the section “Dose Constraints.”
Creation of an internal target volume (ITV) with the assistance of a 4D CT scan to account for respiration will depend on the degree of tumor motion and the method of respiratory motion management. Respiratory-gated treatments centered at end exhalation or breath-hold techniques may require little to no adjustment for the ITV. However, if patients are treated free-breathing, consideration should be given to creating an ITV or using a final planning target volume (PTV) margin that accounts for the degree of tumor excursion. PTV expansion is set by individual institutional practice depending on the frequency and type of on-board imaging, respiratory motion management strategy, and the presence of fiducial markers. Our practice is to use respiratory gating in the presence of fiducials, and typically a 3-mm expansion of the GTV is sufficient. If patients are free-breathing with abdominal compression and without fiducials, typical PTV margins may range from 5 to 10 mm (Figure 15.2).
232Liver Metastases and Intrahepatic Cholangiocarcinoma
Principles of simulation and treatment planning for metastatic disease and ICC are similar to those for HCC. Any diagnostic CT and MRI scans should be used in conjunction with all treatment-planning scans for target delineation. However, with the exception of patients with metastatic neuroendocrine tumors or other tumors that demonstrate arterial enhancement, an arterial-phase CT scan is not routinely performed in our institutional practice. In addition, a PET scan may be useful to further identify the extent of disease and aid in target delineation (80).
DOSE PRESCRIPTION AND FRACTIONATION
Various dose prescription and fractionation schedules have been reported for SBRT for primary liver malignances, and no consensus guidelines exist for selecting an optimal dose. Ablative doses can be achieved using a variety of hypofractionated schedules ranging from one to 15 fractions. The prescription dose is often limited by the volume of normal liver, underlying liver function, and adjacent normal tissue constraints. Four published prospective studies have investigated SBRT for HCC, with dose prescriptions ranging from 36 to 60 Gy in three to six fractions (13–16). Investigators from Indiana University and the University of Colorado conducted a phase I dose escalation study, successfully treating HCC to 48 Gy in three fractions for patients with CP A, but determined that 40 Gy in five fractions was the maximally tolerated dose for patients with CP class B (CP B) (13). The currently open NRG Oncology (consortium made up of the National Surgical Adjuvant Breast and Bowel Project [NSABP], RTOG, and the Gynecologic Oncology Group [GOG]) 1112 trial adapts the prescription dose, which ranges from 27.5 to 50 Gy, in five fractions on the basis of the dose to the normal liver (81). At our institution, the prescription liver SBRT for patients with CP A cirrhosis and small peripheral primary tumors is 45 Gy in three fractions if dose constraints are met. For lesions near bowel or central liver or for larger lesions, we typically use a five-fraction regimen ranging from 30 to 50 Gy. For patients with CP B liver function, a five-fraction regimen ranging from 25 to 40 Gy is used. Investigators at Massachusetts General Hospital and MD Anderson conducted a phase II trial of hypofractionated ablative proton RT for primary liver tumors, using a 15-fraction regimen to 67.5 GyE for small peripheral tumors or 58.05 GyE for central tumors (18).
Principles of dose prescription and fractionation of SBRT are similar for metastatic tumors although, in general, higher biologically effective doses (BEDs) have been used primarily because of the absence of underlying cirrhosis leading to increased liver tolerance. Among other factors, key considerations include tumor histology, number of lesions, and the size of lesions. Data suggest that radioresistant histologies such as melanoma and renal cell carcinoma require doses of 48 to 49 Gy in three fractions (82). Similarly, for colorectal metastases, there is emerging evidence that dose escalation may be appropriate in the lung SBRT setting (83), and data also suggest that a dose of 48 Gy in three fractions may be needed to optimize local control for colorectal liver metastases (84). Other factors include the number and size of lesions and their location. Therefore, selection of final prescription dose and fractionation must strike a balance between maximizing efficacy and sparing adjacent critical structures. Our practice is to use a dose of 45 to 54 Gy in three fractions for peripheral tumors limited in size and number and away from sensitive organs, with higher range doses particularly used for metastases from colorectal carcinoma, renal cell carcinoma, or melanoma. For larger tumors more centrally located or near the bowel, esophagus, stomach, or heart, a dose of 40 to 50 Gy in five fractions is typically used. Surgical placement of biological mesh spacers composed of acellular human dermis has also been shown to sufficiently displace bowel away from tumors to allow for the safe delivery of RT (85).
DOSE CONSTRAINTS
The primary OARs with liver SBRT include the uninvolved liver, heart, chest wall, ribs, kidneys, stomach, duodenum, bowel, spinal cord, and cHBT. For the liver, classic RILD is a syndrome of an acute triad of hepatomegaly, ascites, and elevated ALP followed by the development of anicteric ascites approximately 2 weeks to 4 months after hepatic irradiation described with conventional fractionation (7) but has rarely been reported in SBRT studies. Nonclassic RILD has emerged as a more commonly seen toxicity, described as a fivefold or higher increase in transaminase values (10), a decrease in liver function loosely defined as a 2332-point or higher increase in CP score, reactivation of hepatitis, or any other toxicity not included in the classic RILD syndrome (86). Because nonclassic RILD encompasses a wide range of etiologies and symptoms and has only been recently recognized, appropriate dose constraints as a preventative measure are poorly understood.
The University of Colorado first used the approach of sparing a critical volume of normal liver by ensuring that at least 700 mL of normal liver was the minimum volume that must receive a total dose less than 15 Gy in a phase I trial of SBRT for liver metastases showing essentially no cases of liver toxicity (87, 88). Since then, several SBRT investigators have adopted the critical volume model as their constraint with few toxicity events as long as constraints were met (24, 89–91). Investigators have similarly used a critical volume approach for patients with HCC with a similar constraint of limiting 700 mL of liver receiving greater than 15 Gy. Son et al. proposed sparing 800 mL of liver from receiving greater than 18 Gy in three fractions to reduce hepatic toxicity in patients with CP-Class A cirrhosis (92). Investigators from Princess Margaret used NTCP modeling to adapt the dose of radiation on the basis of predicted risk of liver toxicity and found that the mean liver dose in patients with grade 5 toxicity was higher than in those who did not (median 18.1 Gy vs. 15.4 Gy, p = .02) (16, 12). The NRG 1112 trial allows dose adaption on the basis of the mean liver dose, which is limited to 13 to 17 Gy.
Central liver toxicity is an increasingly recognized late effect of SBRT for liver tumors. Osmundson et al. first proposed delineation of the cHBT by using a 1.5-cm expansion of the portal vein. Retrospectively analyzing 96 patients treated with SBRT at Stanford University, investigators found a significant association of cHBT and BED after converting dose fractionation schemes using the standard linear quadratic model (α/β = 10) (78). These findings were further validated in an expanded analysis in which investigators showed that central liver toxicity and dose to cHBT were significantly associated with primary liver tumors (HCC and ICC) but not with metastases. In addition, a more detailed analysis of dose–response led to the proposed constraints to the cHBT of V40 less than 37 mL and V30 less than 45 mL (79).
The stomach, duodenum, and remainder of the bowel represent other critical OARs to which serious long-term toxicity such as ulceration and perforation may be possible if careful attention is not given during treatment planning. Dose constraints to these organs include Dmax less than 40 Gy, V25 less than 9 mL, V30 less than 5 mL, and V35 less than 1 mL. To minimize the risk of late cardiotoxicity, the heart constraints used are Dmean less than 12 Gy and V15 less than 10%. To minimize the risk of kidney failure, the kidney dose should be kidney V5 less than 50%. To minimize the risk of chest wall pain and rib fracture, the chest wall dose is limited to V30 less than 30 mL, and ribs D2mL less than 27 Gy. The aforementioned dose constraints and other organs are summarized in Table 15.2. Pollom et al. have recently conducted a review of these dose constraints (93).
TREATMENT PLANNING
Our current institutional practice is to plan cases with liver SBRT delivered on gantry-based linear accelerators with volumetric-modulated arc therapy (VMAT) using one partial or full arc with 10 MV multileaf collimator (MLC)–shaped flattening filter-free (FFF) beam. FFF beam allows for creation of ablative heterogeneous dose distribution within the target and sharp dose falloff from target to surrounding normal tissue. In addition, the high dose rate of FFF beams makes the SBRT treatment delivery very efficient. Small peripheral tumors away from sensitive critical structures could potentially be treated with multibeam three-dimensional conformal plans, although inverse planning is generally needed to achieve the high degree of conformality necessary to safely deliver ablative doses. Dose calculation is performed using convolution/superposition Analytical Anisotropic Algorithm (AAA), Eclipse™ (Varian Medical Systems, Palo Alto, California) in the absence of large low-density heterogeneities, or the more precise Acuros XB algorithm, Eclipse, which uses linear Boltzmann transport equations (LBTEs) and more accurately models the electron scatter and lateral scatter disequilibrium near air/bone/tissue interfaces (94).
Treatment planning with CyberKnife® (Accuray, Inc., Sunnyvale, California) also involves inverse planning using pencil beam arrangements to conform to the target. The beams can be shaped by collimators ranging from 5 to 60 mm in diameter, Iris collimator (a 12-sided regular polygon, which can automatically adjust the aperture size), or InCise™ MLC (with maximum field size of 10 cm × 12 cm at 80-cm source-to-axis distance). As with inverse planning on gantry-based linear accelerators, planning for CyberKnife is an iterative optimization process based on meeting a list of set priorities of target coverage, conformality, and sparing of surrounding normal tissues. Dose calculation can be performed via either simple ray-tracing pathlength-correction algorithm or the more accurate but calculation-intensive Monte Carlo algorithm that relies on particle interaction probabilities and subsequent dose depositions derived from clinically measured dosimetric parameters. The use of the ray-tracing algorithm is quite sufficient in mostly homogenous treatment areas, but if the tumor is near the diaphragm, dose calculation with ray tracing is less accurate because of the lack of corrections for changes in electron transport and lateral scatter disequilibrium that might develop in the presence of varying density heterogeneities (95). Hence, liver SBRT plans with treatment targets close to the liver dome should be ideally calculated via the Monte Carlo algorithm.
234TABLE 15.2 Common Institutional Dose Constraints Used for Liver Stereotactic Body Radiation Therapy
Organ at Risk | Dose Constraint |
Liver—noncirrhotic | ≥700 cm3 of uninvolved liver <15 Gy (three fractions) ≥700 cm3 of uninvolved liver <21 Gy (five fractions) |
Liver—cirrhotic | Child–Pugh class A ≥700 cm3 of uninvolved liver <15 Gy (in three or five fractions) Mean liver dose <15 Gy (in three or five fractions) Child–Pugh class B ≥700 cm3 of uninvolved liver <15 Gy (five fractions) ≥500 cm3 of uninvolved liver <7 Gy (five fractions) Mean liver dose <10 Gy (in five fractions) |
Central hepatobiliary tree | V40 <37 cm3 and V30 <45 cm3 (five fractions) |
Stomach, duodenum, bowel | Dmax <40 Gy, V25 <9 cm3, V30 <5 cm3, V35 <1 cm3 (five fractions) V30 <1 cm3, V24.5 <2 cm3 (three fractions) |
Heart | Dmean <12 Gy, V15 <10% |
Kidney | V5 <50% |
Chest wall | V30 <30 cm3 (recommended) |
Ribs | D2mL <27 Gy (recommended |
Spinal cord | Dmax <20 Gy (three fractions) Dmax <15 Gy (three fractions) |
Dmax, maximum dose; Dmean, mean dose; Dn mL, dose received by n mL of tissue; Vn, volume of tissue receiving n Gray.
Effective plan evaluation should be consistent and follow a reproducible algorithm set by the individual practitioner (96). Communication between physician and dosimetry staff is essential because of the iterative nature of plan improvement. The goals of treatment planning include a prescription dose tightly conforming to the target volume, an ablative and nonuniform dose distribution within the PTV with highest doses within the GTV, a rapid dose falloff in all directions outside the target volume, high dose gradients between adjacent normal tissue and the target, and the highest possible dose reduction to OARs (97).
Essential to treatment planning is meticulous evaluation of the dose–volume histogram and isodose levels paying specific attention to target coverage, dose to OARs, dose homogeneity, and dose falloff gradients (Figures 15.3 and 15.4). Typically, the goal is to have at least 95% of the target volume covered by 100% of the prescribed dose. Given rapid dose falloff outside of target volume, global maximum doses of 110% to 130% of prescription dose are acceptable but should be located within the target volume, preferably within the GTV. Prescription doses may be limited by normal tissue constraints, in which case the maximum prescription dose that allows meeting these constraints is usually chosen. An alternative approach is to maintain the desired prescription dose but place higher priority on meeting OAR constraints and thereby reduce target coverage to less than 95%. There are no data to clearly support either one approach over the other, but practitioners should be mindful of the minimum PTV and GTV dose.
235
FIGURE 15.3 Evaluation of a gantry-based SBRT plan for a 70-year-old woman with small-cell lung cancer treated to a single metastatic site in the liver. (A) Axial images of dose distribution and (B) dose–volume histogram for uninvolved liver. Mean uninvolved liver dose was 7.3 Gy; 904 cm3 (≥700 cm3) of uninvolved liver received less than 15 Gy, and 749 cm3 (≥500 cm3) of uninvolved liver received less than 7 Gy. The patient was treated with three fractions of 15 Gy for a total dose of 45 Gy.
SBRT, stereotactic body radiation therapy.
FIGURE 15.4 Axial images displaying isodose levels for a 62-year-old woman with metastatic sigmoid carcinoma treated with SBRT for two metastatic lesions in her liver. She was treated with three fractions of 15 Gy daily for a total dose of 45 Gy. Isodose lines spatially represent dose distributions as a percentage of prescription dose. In this figure, isodose lines shown include red (100%), green (80%), yellow (60%), purple (40%), blue (25%), and dark blue (10%). Red color wash is a contour of the planning target volume.
SBRT, stereotactic body radiation therapy.
236
FIGURE 15.5 Gantry-based SBRT treatment of HCC. (A) Axial, (B) coronal, and (C) sagittal images of dose distribution and (D) three-dimensional surface rendering of a SBRT plan delivered via a gantry-based system. The patient is a 63-year-old man with a history of hepatitis C, cirrhosis, and hepatocellular carcinoma after transarterial chemoembolization. The patient was treated to a dose of 45 Gy in five fractions.
HCC, hepatocellular carcinoma; SBRT, stereotactic body radiation therapy.
TREATMENT DELIVERY
In this section, we outline two methods of treatment delivery: gantry-based (i.e., Varian, Electa, Siemens) and robotic-based (i.e., CyberKnife) linear accelerator platforms. Although each offers its own dosimetric advantages (98), data about head-to-head efficacy and toxicity with respect to treatment of the liver are lacking. Furthermore, both platforms have different methods to account for tumor intrafractional motion to allow a reduction in treated volumes. These methods include gating (99), tracking (100), and fixation (101). Gantry-based systems use phase or amplitude gating via commercially available motion monitoring devices such as Real-Time Position Management™ (RPM) system (Varian Medical Systems), Response™ gating control (Elekta AB, Stockholm, Sweden), or ANZAI gating system (ANZAI Medical Co., Ltd., Tokyo, Japan) and/or inhibition such as abdominal compression or breath hold. In contrast to gating and breath-holding techniques on gantry-based delivery platforms that deliver radiation during a portion of the tumor motion, the robotic arm–based platform such as CyberKnife (Accuray, Inc.) is the only system capable of respiratory tracking (Synchrony™) that moves the beam to follow the motion of the tumor, thus maintaining constant beam’s-eye view of the tumor throughout the respiratory cycle and delivery of radiation throughout.
Gantry-Based Delivery
Primary components of gantry-based delivery systems include a multienergy S-band linear accelerator with gantry, table, and collimator rotating around the isocenter, with kV and MV on-board imaging systems for image guidance. Treatment can be delivered using VMAT or multiple isocentric coplanar or noncoplanar static beam arrangements (102) (Figure 15.5). The exact method of target verification and respiratory motion management is institution-dependent. At Stanford, the institutional practice is to first verify alignment of bony anatomy and the fiducial markers using orthogonal kV and fluoroscopic imaging. Amplitude respiratory gating at end exhalation is used, with the amplitude and gating window manually set on the basis of the position of the fiducial markers relative to their position on the digitally reconstructed radiograph (DRR) such that the “beam-on” occurs when the fiducials move inside the projected fiducial contour on fluoroscopy (Figure 15.6). Beam-level kV imaging is taken prior to each “beam-on” cycle to verify the positioning of tumor markers during the treatment delivery (Figure 15.7).
Robotic Arm–Based Delivery
Primary components of robotic arm–based delivery system include a 6-MV compact X-band linear accelerator attached to a robotic articulating manipulator, with two stereoscopic x-ray sources for image guidance. Such systems do not rely on a coordinate-based isocenter to deliver treatment, but instead deliver a large number of noncoplanar beams from nodes and angles automatically selected from the predefined path (Figures 15.8 and 15.9). Image guidance is based on implanted fiducial markers through the use of orthogonal x-ray images every 10 to 30 seconds. The Synchrony (Accuray, Inc.) tracking system accounts for respiratory motion by tracking the predicted position of the fiducial markers on the basis of the correlation model built from several sets of orthogonal x-rays that are then associated with the position of infrared light-emitting diodes on the chest wall. This predictive model is continuously updated with a new pair of x-rays taken every 90 seconds during the treatment delivery process. The patient breathes freely during the treatment delivery, and the robotic arm moves the gantry to follow the tumor motion predicted by the correlation model that is constantly automatically updated with no user intervention required.
237FIGURE 15.6 Motion management during liver SBRT treatment. (A) Anterior–posterior digitally reconstructed radiograph demonstrating radiopaque fiducials optimally within the treatment position at end expiration (green circles). Fiducials serve as a surrogate for breathing and thus move during a patient’s respiratory cycle. (B) Fiducial positioning during “beam-on” with yellow circles and (C) fiducial positioning during “beam-off” with green circles.
SBRT, stereotactic body radiation therapy.
FIGURE 15.7 Respiratory-gated VMAT treatment. Beam-level kV imaging is taken prior to each “beam-on” cycle to verify fiducial positioning during the treatment arc. (A–D) Screenshots of beam-level imaging at different gantry angles along the VMAT gated arc path.
VMAT, volumetric-modulated arc therapy.
FIGURE 15.8 Three-dimensional rendering of 167 non–isocenter-based treatment beams used to treat a patient with metastatic disease to the liver.
FIGURE 15.9 Robotic arm–based treatment of metastatic disease. Axial, sagittal, and coronal dose distribution for a patient treated with SBRT via a robotic arm–based system. The patient is an 81-year-old man with a history of metastatic colon cancer treated with a single fraction of 25 Gy using 167 total nonzero beams prescribed to the 79% isodose line.
SBRT, stereotactic body radiation therapy.
RESPONSE ASSESSMENT AND SURVEILLANCE
Current guidelines with respect to the optimal response assessment and surveillance of patients with HCC treated with SBRT are lacking. In fact, such data are limited even for patients who have undergone other, longer established locoregional therapies such as surgical resection. Given that both surgery and SBRT represent a locoregional 238modality, extrapolation from the recommendations from the surgical population is reasonable.
As such, current expert consensus panels recommend ongoing posttreatment surveillance (103). Imaging should consist of high-quality, triphasic, contrast-enhanced CT or MR-based imaging with the first assessment as early as 4 weeks following locoregional therapy to establish a new baseline (104) (Figure 15.10). However, the initial post-SBRT imaging can be obtained at 3 months to allow resolution of radiation inflammation. Thereafter, repeat imaging is recommended every 3 to 6 months for the initial 2 years after treatment. This duration of interval surveillance is based on observational data, which were used to estimate the anticipated growth rates of HCC (105, 106). After the first 2 years, imaging can be obtained every 6 to 12 months or a liver ultrasound every 6 months may be recommended per the accepted 2010 American Association for the Study of Liver Diseases (AASLD) guideline on HCC surveillance (107). If initially elevated, laboratory testing with serum alpha-fetoprotein (AFP) levels should be obtained every 3 months for the first 2 years, and then every 6 to 12 months thereafter. In the event of suspicious imaging or laboratory findings, additional workup should be initiated per the algorithm for de novo disease.
FIGURE 15.10 Serial follow-up imaging of a liver lesion treated with SBRT. The patient was a 70-year-old female with a 1.3-cm lesion of metastatic small-cell lung cancer to segment eight of the liver treated with SBRT to 45 Gy in three fractions. (A) Axial CT at treatment planning, (B) axial CT at 3 months post-SBRT showing partial response, and (C) axial CT at 9 months post-SBRT showing complete response.
SBRT, stereotactic body radiation therapy.
As a general rule in HCC, the lack of contrast uptake on CT or MRI signals a favorable treatment effect to tumor ablation (108). The desired outcome on follow-up imaging after SBRT is to produce a nonenhancing ablated zone encompassing the entire tumor. However, persistent contrast uptake and arterial vascular enhancement within the tumor followed by contrast washout in the delayed phase may not necessarily represent treatment failure. Any areas of apparent persistent enhancement should be serially monitored, as a delayed tumor response has been documented for HCC treated via SBRT (108–110).
In general, for primary and metastatic liver tumors, definitions of stable disease, partial response, or complete response use Response Evaluation Criteria in Solid Tumors (RECIST) on the basis of extent of local control, the methodology of response assessment used in NRG 1112. One such study on 277 tumors showed that radiographic complete response rates at 3, 6, and 12 months posttreatment were 24%, 67%, and 71%, respectively, with a small fraction of tumors showing response greater than 2 years posttreatment (111). Modified RECIST have been proposed to account for evaluating treated lesions that do not necessarily change in size (112). Thus, the determination of disease status is based on the response of the viable enhancing portion of the target lesion, and the definition of local control can be considered the absence of progressive disease of enhancing viable tumor.
Follow-up imaging can be challenging to read as regions of nontumorous hepatic parenchyma treated with SBRT will have evidence of arterial hypervascularity in up to 50% of patients at 6 months posttreatment (113), which can serve as a potential source for false-positive recurrences. The key difference between nontumorous enhancement and viable tumor is that normal liver parenchyma after SBRT will have persistent contrast enhancement in the delayed phase, whereas tumor will undergo delayed washout.
Finally, it is also important to keep in mind that patients with HCC may have underlying liver disease that itself can cause significant morbidity and mortality. Such patients must have the appropriate surveillance under a multidisciplinary team for progression of liver dysfunction and esophageal varices in addition to their standard post-SBRT assessments.
For metastatic lesions, single-venous phase-contrast CT is sufficient for surveillance imaging on a similar schedule. Caution should be used to avoid confusing the hypodense appearance of the adjacent liver parenchyma within the radiation penumbra with progression of the target lesion (Figure 15.11). In addition, PET imaging may also be useful to determine residual active disease for lesions that are initially FDG-avid. It is important to know that metabolic response may be delayed for several months, with one study showing that the time for maximum standardized uptake value (SUVmax) to reduce by half was 3.8 months with median SUVmax of 2.6 at 7 months (82).
239
FIGURE 15.11 Imaging of benign liver changes following SBRT demonstrating liver parenchymal changes that may mimic tumor progression. (A) SBRT plan showing isodose distribution, (B) CT 3 months after SBRT showing area of hypodensity that approximates the 40% prescription isodose line, and (C) CT 7 months after SBRT showing resolution of the hypodensity changes seen earlier.
CT, computed tomography; SBRT, stereotactic body radiation therapy.
OUTCOMES
There are several retrospective and prospective studies that detail patient outcomes following liver SBRT for unresectable HCC, ICC, and liver metastases from other primary sites (Table 15.3). However, little data are available directly comparing SBRT with other local treatment modalities for unresectable disease such as RFA or TACE. It should be noted that patients with liver metastases often have better liver function than patients with HCC, and the radiosensitivity of these tumors may be different. Many early studies pooled results from patients with HCC, liver metastases, and even ICC, making it difficult to compare across studies and treatment approaches.
Hepatocellular Carcinoma
Multiple retrospective studies have demonstrated excellent local control of HCC with variable overall survival (OS) after SBRT, depending mostly on the comorbidities of the treatment population (114). Reported local control has ranged from as low at 51% at 3 years for larger tumors treated with a median of 45 Gy in three to five fractions (115) to as high as 91% at 3 years with 35 to 40 Gy in five fractions for smaller tumors (116).
Four published prospective studies have investigated SBRT for HCC. Cárdenes et al. performed a phase I dose escalation trial using 36 to 48 Gy in three fractions for CP A and 40 Gy in five fractions for CP B patients with primary HCC and reported 100% local control at a median follow-up of 24 months with 2-year survival of 60% (13). In an update of their combined phase I/phase II trial, the authors reported a 2-year local control of 90% and OS of 67% with the majority of the patients receiving 48 Gy in three fractions for CP A and 40 Gy in five fractions for CP B (14). Bujold et al. treated patients with HCC using 24 to 53 Gy in six fractions and reported 1-year local control of 87% with complete response in 11% of patients (16). Median survival was 17.0 months. Kang et al. treated patients with inoperable HCC with incomplete response to TACE with 42 to 60 Gy in three fractions (15). Of these patients, 38.3% had a complete response and 38.3% had a partial response. Two-year local control was 94.6% with 68.7% OS.
Previous studies have shown that percutaneous ablation outperforms transarterial chemoablation, particularly for smaller HCC tumors (117, 118). Prospective studies evaluating percutaneous ablation with RFA, percutaneous ethanol injection, percutaneous acetic acid injection, or microwave ablation have reported local control rates of 47% to 88.6% with 3-year OS of 33% to 77.8% (65). Although many patients with HCC included on SBRT trials to date either have received other treatments earlier or are unsuitable for typical local therapies, the current data suggest that SBRT compares favorably with other local treatment options in terms of local control and OS.
Wahl et al. retrospectively compared SBRT with RFA for inoperable HCC (29). Despite patients treated with SBRT having worse liver function prior to treatment, they reported 2-year freedom from local progression of 80.2% for RFA and 83.8% for SBRT and OS of 53% for RFA and 46% for SBRT. Importantly, for tumors of 2 cm or more, SBRT had significantly improved freedom from local progression compared with RFA, suggesting that SBRT is more effective for larger tumors. However, this study used the original RECIST, which may not account for tumor necrosis and may affect the assessment of local progression (119). Overall, these data support the use of SBRT as primary treatment for inoperable HCC, and further randomized clinical trials will be needed to establish the first-line local therapy for inoperable HCC.
240
Data are emerging comparing SBRT with TACE. Sapir et al. retrospectively compared outcomes of patients treated at the University of Michigan with SBRT (125 patients with 173 lesions) and TACE (84 patients with 114 lesions). The local control for SBRT versus TACE was 96.5% versus 47% at 1 year and 91% versus 23% at 2 years (p < .001). Patients treated with SBRT also had higher rates of freedom from hepatic progression at 1 year (56.5% vs. 36%) and 2 years (27% vs. 11%) compared with patients treated with TACE (p < .001) (69). In a prospective randomized trial, investigators at Loma Linda University compared proton RT (70.2 Gy in 15 fractions) with TACE (17). Although only 69 patients were randomized, the 2-year local control was 88% for RT and 45% for TACE (p = .06), and the 2-year progression-free survival was 48% for RT and 31% for TACE (p = .06).
241SBRT can also be used to “bridge” patients to liver transplantation, as up to 30% of patients awaiting transplantation are removed from the list because of progressive disease (38). O’Connor et al. reported on the long-term outcomes of SBRT as a bridge to orthotopic liver transplantation in patients with HCC. With a median SBRT dose of 51 Gy (range 33–54 Gy) in three fractions, both the 5-year overall and disease-free survival rates were 100%. Explant pathology revealed a complete response rate in 27% of patients, with the remaining patients having tumors that decreased or remained stable in size (120). Barry et al. retrospectively reviewed outcomes in 400 patients with HCC receiving SBRT as a bridge for liver transplant. With a median SBRT dose of 36 Gy in six fractions, 5-year overall and disease-free survival in patients who went on to have transplant were 76% and 79%, respectively (38). There was no increase in operative morbidity during the time of transplant, and the authors concluded that SBRT can be a safe and effective bridge therapy to liver transplant.
Investigators at Massachusetts General Hospital and MD Anderson reported results of phase II prospective study of 92 patients (83 analyzable patients) with HCC (n = 44) or ICC (n = 39) treated with proton RT (58.05 GyE for central tumors within 2 cm of the porta hepatis or 67.5 GyE for peripheral tumors >2 cm from the porta hepatis), with a median dose delivered of 58 Gy. The median tumor size was 5 cm for HCC and 6 cm for ICC, and approximately 29% of patients had tumor vascular thrombus. Despite the locally advanced disease of these patients, the 2-year local control was 94.8% for HCC and 94.1% for ICC (18).
The selection of protons versus photons remains a subject of controversy given the excellent local control seen with both proton- and photon-based therapy for HCC. The proton series show impressive OS and it remains unclear if this is because of patient selection or improved hepatic function because of a yet unclarified dose–volume relationship with increasing CP score. A randomized trial is planned directly comparing protons with photons for HCC (NRG GI-003).
Intrahepatic Cholangiocarcinoma
Liver SBRT is indicated for the management of unresectable ICC. The available evidence is limited primarily to single-institution retrospective studies. The Mayo Clinic reported on 10 patients with unresectable or recurrent advanced cholangiocarcinoma who underwent SBRT to a median dose of 55 Gy in three or five fractions. One-year freedom from distant progression and OS were 31% and 73%, respectively, with one case of grade 3 biliary stenosis and one case of grade 5 liver failure (121). O’Connor et al. reported the Baylor University experience of 12 patients with unresectable HCC treated with SBRT to a median dose of 24 Gy in three fractions, and found 75% rate of local control with 25% complete response rate for patients with follow-up imaging, with 25% rate of acute toxicity (all grade 1) (40). Sandler et al. reported the University of California–Los Angeles (UCLA) experience of 25 patients with unresectable extrahepatic disease and six patients with ICC who underwent SBRT to a median dose of 40 Gy in five fractions. Median time to progression and OS were 16.8 and 31.3 months, respectively, at the cost of 77% grades 1 to 2 toxicity and 16% grade 3 or higher toxicity. Four patients actually received SBRT as bridge therapy and eventually underwent liver transplantation (122). Overall, the evidence suggests that SBRT may be a promising 242option for properly selected patients with unresectable or recurrent cholangiocarcinoma, either definitively for local control or as a bridge to liver transplantation.
As mentioned earlier, a prospective trial of proton RT to a median dose of 58 GyE resulted in a 2-year local control of 94.1% (18). Investigators at MD Anderson reported results of dose-escalated EBRT for unresectable ICC in 79 patients (123). The median tumor size was 7.9 cm (range: 2.2–17). Using a median dose of 58.05 Gy (range: 35–100) in three to 30 fractions and a median BED of 80.5 Gy (range: 43.75–180 Gy), the investigators found 3-year local control of 78% for BED more than 80.5 Gy compared with 45% for BED of 80.5 Gy or less (p = .04). In addition, 3-year OS was improved with higher BED compared with that with lower BED (73% vs. 38%, p = .017).
On the basis of these results, a randomized trial has been initiated to evaluate whether the effect on local control seen with high-dose RT translates into improvements in OS. In NRG GI-001, patients are randomized following chemotherapy to high-dose 15-fraction RT versus observation (124).
Liver Metastases
To date, no randomized phase III data have been published on SBRT for liver metastases, but there are several retrospective and prospective clinical studies. Most studies have combined multiple different tumor histologies, with colon cancer, lung cancer, and breast cancer being the most frequently represented. Studies to date have generally limited treatment to patients with five or fewer liver metastases. In prospective studies, reported local control has ranged from 68% at 18 months with 14 to 26 Gy in one fraction (19) to 92% at 2 years with 36 to 60 Gy in three fractions (21). Tumor size appears to be an important factor. Rusthoven et al. reported 2-year local control of 100% for tumors 3 cm or less compared with that of 77% for tumors greater than 3 cm (21). Similarly, Lee et al. found that local control was improved with smaller volume tumors less than 75.2 mL and higher delivered dose on univariate analysis (4). In contrast, Scorsetti et al. did not find any difference in local control rates for using the cutoff established by Rusthoven et al. when using 75 Gy in three fractions (89). There is data suggesting that local control is worse for colorectal metastases (125, 126).
In prospective trials, OS varied from 67% at 1 year following 45 Gy in three fractions for CRC metastases (20) to 83.5% at 1 year following 75 Gy in three fractions for mixed histologies (89). Scorsetti et al. did not find any association between primary tumor site or prior treatments and OS (89). Hoyer et al. found improved OS in patients with metachronous metastases or largest metastasis less than 35 mm (20). Rusthoven et al. reported worse median survival for tumors from the lung, ovaries, and noncolorectal gastrointestinal sites (12 months) versus breast, colorectal, renal, carcinoid, gastrointestinal stromal tumors, and sarcomas (32 months) (21). Similar to HCC, there are more data available for percutaneous ablation of liver metastases with OS up to 33% at 5 years in select patients following RFA (127). Long-term follow-up after SBRT and prospective randomized trials will be necessary to determine the optimal first-line nonsurgical approach.
QUALITY OF LIFE
Few studies have focused specifically on the effect of liver SBRT on quality of life for patients. Given the poor long-term outcome in the majority of patients with HCC and liver metastases, quality of life is critically important when evaluating treatment options. A recent value of information analysis suggested that additional studies evaluating quality of life after liver-directed therapies would provide the best information for guiding treatment decisions in patients with liver tumors (128). Because RT is a noninvasive procedure, its inherent advantage is to reduce acute morbidity and mortality. For example, a prospective randomized trial of proton RT versus TACE with 69 patients with HCC revealed that proton RT had significantly fewer hospitalization days within 1 month of treatment compared with TACE (24 days vs. 166 days, p < .001) (17).
In patients with HCC, liver metastases, or ICC, Klein et al. found that liver SBRT did not affect overall quality of life on the basis of both general and liver-specific quality of life questionnaires (129). Appetite loss and fatigue significantly worsened 1 month after treatment, but recovered by 3 months. Higher baseline quality of life scores were associated with improved survival. Méndez Romero et al. reported similar results with mean quality of life scores trending toward improvement compared with baseline after treatment (130). These results are promising and suggest that SBRT is well tolerated and may delay deterioration of quality of life secondary to liver tumor progression. Additional studies are needed to establish the baseline quality of life in these patients and more 243importantly to compare with other established therapies that may be associated with more invasiveness and thus more morbidity.
SAFETY CONSIDERATIONS
For liver metastases, most studies have demonstrated 1% to 10% grade 3 or higher toxicity associated with SBRT (131). Although transient elevations in hepatic transaminases are common following SBRT, most prospective studies have reported no or rare incidence of radiation-induced liver disease (RILD) (4, 21, 89). The highest rate of grade 3 liver toxicity was reported by van der Pool et al. whose study found that two of their 20 patients developed grade 3 liver toxicity with liver enzyme elevation following three fractions of 12.5 to 15 Gy per fraction (22). However, in prospective studies, the rate of RILD appears to be less than 1% following SBRT for liver metastases.
Given the frequency of preexisting liver disease in patients with HCC, it is difficult to distinguish RILD from worsening of previous liver disease unrelated to SBRT. However, worse baseline liver function appears to predispose to liver toxicity. In the Cárdenes et al. phase I dose escalation study for primary HCC, two CP B patients developed grade 3 hepatic toxicity after 42 Gy in three fractions, and their protocol was amended to 40 Gy in five fractions for CP B patients with only one additional patient developing progressive liver failure (13). Andolino et al. reported that 20% of patients developed progression of their CP class after SBRT within 3 months of treatment, and two of the 56 patients who completed treatment died from progressive liver failure within 3.5 and 4 months (14). There was no association between toxicity and the dose delivered to normal liver. Although Bujold et al. reported no classic RILD in their patients, they observed a decline in CP class in 29% of patients at 3 months and in 6% at 12 months that was not related to progression of their HCC (16).
The most common extrahepatic complications result from lesions close to the bowel and chest wall. Kang et al. reported that two of their 47 patients with inoperable HCC developed gastric ulcer perforation (15). On retrospective review of their data, they identified that three of five patients with preexisting gastroduodenal ulcers developed severe GI toxicities after SBRT, suggesting that preexisting ulcers are a significant risk factor for GI toxicity. They suggested that esophagogastroduodenoscopy (EGD) could be beneficial to determine the presence of ulcers if tumors are located near the stomach or duodenum. Lee et al. reported grade 2 nontraumatic rib fractures in two patients treated for liver metastases after SBRT associated with a maximum dose to 0.5 mL of rib of 51.8 and 66.2 Gy in six fractions (4). Rusthoven et al. reported that a patient treated with 60 Gy in three fractions for an inferior-lateral right lobe carcinoid metastases developed grade 3 soft tissue breakdown requiring surgical debridement (21). On review of the patient’s plan, this area had received 48 Gy. These toxicities emphasize the importance of careful planning and patient selection to limit dose to surrounding structures.
Other considerations in dose prescription and fractionation include exacerbated toxicity in the setting prior to local therapies in the same area, particularly transarterial radioembolization, where data suggest increased risk of severe liver toxicity when embolization and EBRT are combined (132). In addition, there is emerging evidence that combining hypofractionation with targeted agents against vascular endothelial growth factor (VEGF) may be associated with unexpected and severe gastrointestinal toxicity (133), and thus caution should be applied in this setting until further understanding of this interaction is available.
Physicians should also be aware of chemotherapy-associated liver injury, particularly in patients who undergo extensive chemotherapy for colorectal liver metastases. The chemotherapy regimen determines the pattern of hepatic parenchymal injury—oxaliplatin-based regimens are associated with grade 2 or higher sinusoidal injury and irinotecan-based regimens are associated with steatohepatitis (134). Such injuries may carry implications in the functional reserve of remaining liver in patients who undergo SBRT to focal lesions, although data are currently lacking.
CONCLUSIONS
SBRT has become an increasingly adopted modality for treatment of patients with primary and secondary liver tumors. The highly conformal and precise nature of SBRT allows for safe dose escalation leading to improved outcomes without increased rates of serious toxicities such as radiation-induced liver disease. Finally, combining SBRT with other locoregional and systemic treatment modalities may lead to further improvements in disease outcomes, and these approaches are under active investigation.
244SUMMARY OF DOSE CONSTRAINTS (TABLE 15.4)
TABLE 15.4 Dose Constraints for Liver Primaries and Metastases Using SBRT
Liver Metastases or Hepatocellular Carcinoma | 60–75 Gy in Four to Five Fractions |
Critical Structures | Suggested Dose Limits |
Liver | ≥700 mL <21 Gy (five fractions) ≥700 mL <15 Gy (three fractions) |
Mean liver dose | ≤15.4 Gy |
Stomach Duodenum Bowel | Dmax <40 Gy V25 <9 mL V30 <5 mL V35 <1 mL |
Kidney | V5 <50 Gy |
Kidney (ipsilateral) | V12.3Gy <130 mL |
Chest wall | V30 <30 mL D2 mL <27 Gy |
Heart | Dmean <12 Gy V15 <10% |
Spinal cord | Dmax <15 Gy (three fractions) |
SBRT, stereotactic body radiation therapy.
REFERENCES
1. Mornex F, Girard N, Beziat C, et al. Feasibility and efficacy of high-dose three-dimensional-conformal radiotherapy in cirrhotic patients with small-size hepatocellular carcinoma non-eligible for curative therapies: mature results of the French Phase II RTF-1 trial. Int J Radiat Oncol Biol Phys. 2006; 66(4):1152-1158. doi: 10.1016/j.ijrobp.2006.06.015
2. Seong J, Lee IJ, Shim SJ, et al. A multicenter retrospective cohort study of practice patterns and clinical outcome on radiotherapy for hepatocellular carcinoma in Korea. Liver Int. 2009;29(2):147-152. doi: 10.1111/j.1478-3231.2008.01873.x
3. Liang SX, Zhu XD, Lu HJ, et al. Hypofractionated three-dimensional conformal radiation therapy for primary liver carcinoma. Cancer. 2005;103(10):2181-2188. doi: 10.1002/cncr.21012
4. Lee MT, Kim JJ, Dinniwell R, et al. Phase I study of individualized stereotactic body radiotherapy of liver metastases. J Clin Oncol. 2009;27(10):1585-1591. doi: 10.1200/JCO.2008.20.0600
5. Ingold JA, Reed GB, Kaplan HS, et al. Radiation hepatitis. Am J Roentgenol Radium Ther Nucl Med. 1965;93:200-208. PubMed PMID: 14243011.
6. Meyer J. Stereotactic radiation therapy for hepatic malignancies. Minerva Gastroenterol Dietol. 2016;62(4):305-315. PubMed PMID: 27681222.
7. Lawrence TS, Robertson JM, Anscher MS, et al. Hepatic toxicity resulting from cancer treatment. Int J Radiat Oncol Biol Phys. 1995;31(5):1237-1248. doi: 10.1016/0360-3016(94)00418-K
8. Czito BG, Meyer JJ, Willett CG. Overview of gastrointestinal toxicity of radiation therapy: upto date [Internet]. 2018. Available at: https://www-uptodate-com.laneproxy.stanford.edu/contents/overview-of-gastrointestinal-toxicity-of-radiation-therapy
9. Huang Y, Chen SW, Fan CC, et al. Clinical parameters for predicting radiation-induced liver disease after intrahepatic reirradiation for hepatocellular carcinoma. Radiat Oncol. 2016;11:89. doi: 10.1186/s13014-016-0663-1
10. Cheng JC, Wu JK, Huang CM, et al. Radiation-induced liver disease after three-dimensional conformal radiotherapy for patients with hepatocellular carcinoma: dosimetric analysis and implication. Int J Radiat Oncol Biol Phys. 2002; 54(1):156-162. PubMed PMID: 12182986.
11. Blomgren H, Lax I, Näslund I, et al. Stereotactic high dose fraction radiation therapy of extracranial tumors using an accelerator: clinical experience of the first thirty-one patients. Acta Oncol. 1995;34(6):861-870. PubMed PMID: 7576756.
12. Tse RV, Hawkins M, Lockwood G, et al. Phase I study of individualized stereotactic body radiotherapy for hepatocellular carcinoma and intrahepatic cholangiocarcinoma. J Clin Oncol. 2008;26(4):657-664. doi: 10.1200/JCO.2007.14.3529
13. Cárdenes HR, Price TR, Perkins SM, et al. Phase I feasibility trial of stereotactic body radiation therapy for primary hepatocellular carcinoma. Clin Transl Oncol. 2010;12(3):218-225. doi: 10.1007/s12094-010-0492-x
14. Andolino DL, Johnson CS, Maluccio M, et al. Stereotactic body radiotherapy for primary hepatocellular carcinoma. Int J Radiat Oncol Biol Phys. 2011;81(4):e447-e453. doi: 10.1016/j.ijrobp.2011.04.011
15. Kang JK, Kim MS, Cho CK, et al. Stereotactic body radiation therapy for inoperable hepatocellular carcinoma as a local salvage treatment after incomplete transarterial chemoembolization. Cancer. 2012;118(21):5424-5431. doi: 10.1002/cncr.27533
16. Bujold A, Massey CA, Kim JJ, et al. Sequential phase I and II trials of stereotactic body radiotherapy for locally advanced hepatocellular carcinoma. J Clin Oncol. 2013;31(13):1631-1639. doi: 10.1200/JCO.2012.44.1659
17. Bush DA, Smith JC, Slater JD, et al. Randomized clinical trial comparing proton beam radiation therapy with transarterial chemoembolization for hepatocellular carcinoma: results of an interim analysis. Int J Radiat Oncol Biol Phys. 2016;95(1):477-482. doi: 10.1016/j.ijrobp.2016.02.027
18. Hong TS, Wo JY, Yeap BY, et al. Multi-institutional phase II study of high-dose hypofractionated proton beam therapy in patients with localized, unresectable hepatocellular carcinoma and intrahepatic cholangiocarcinoma. J Clin Oncol. 2016;34(5):460-468. doi: 10.1200/JCO.2015.64.2710
19. Herfarth KK, Debus J, Wannenmacher M. Stereotactic radiation therapy of liver metastases: update of the initial phase-I/II trial. Front Radiat Ther Oncol. 2004;38:100-105. PubMed PMID: 15458194.
20. Hoyer M, Roed H, Traberg Hansen A, et al. Phase II study on stereotactic body radiotherapy of colorectal metastases. Acta Oncol. 2006;45(7):823-830. doi: 10.1080/02841860600904854
21. Rusthoven KE, Kavanagh BD, Cardenes H, et al. Multi-institutional phase I/II trial of stereotactic body radiation therapy for liver metastases. J Clin Oncol. 2009;27(10): 1572-1578. doi: 10.1200/JCO.2008.19.6329
22. van der Pool AE, Méndez Romero A, Wunderink W, et al. Stereotactic body radiation therapy for colorectal liver metastases. Br J Surg. 2010;97(3):377-382. doi: 10.1002/bjs.6895
23. Goodman KA, Wiegner EA, Maturen KE, et al. Dose-escalation study of single-fraction stereotactic body radiotherapy for liver malignancies. Int J Radiat Oncol Biol Phys. 2010;78(2):486-493. doi: 10.1016/j.ijrobp.2009.08.020
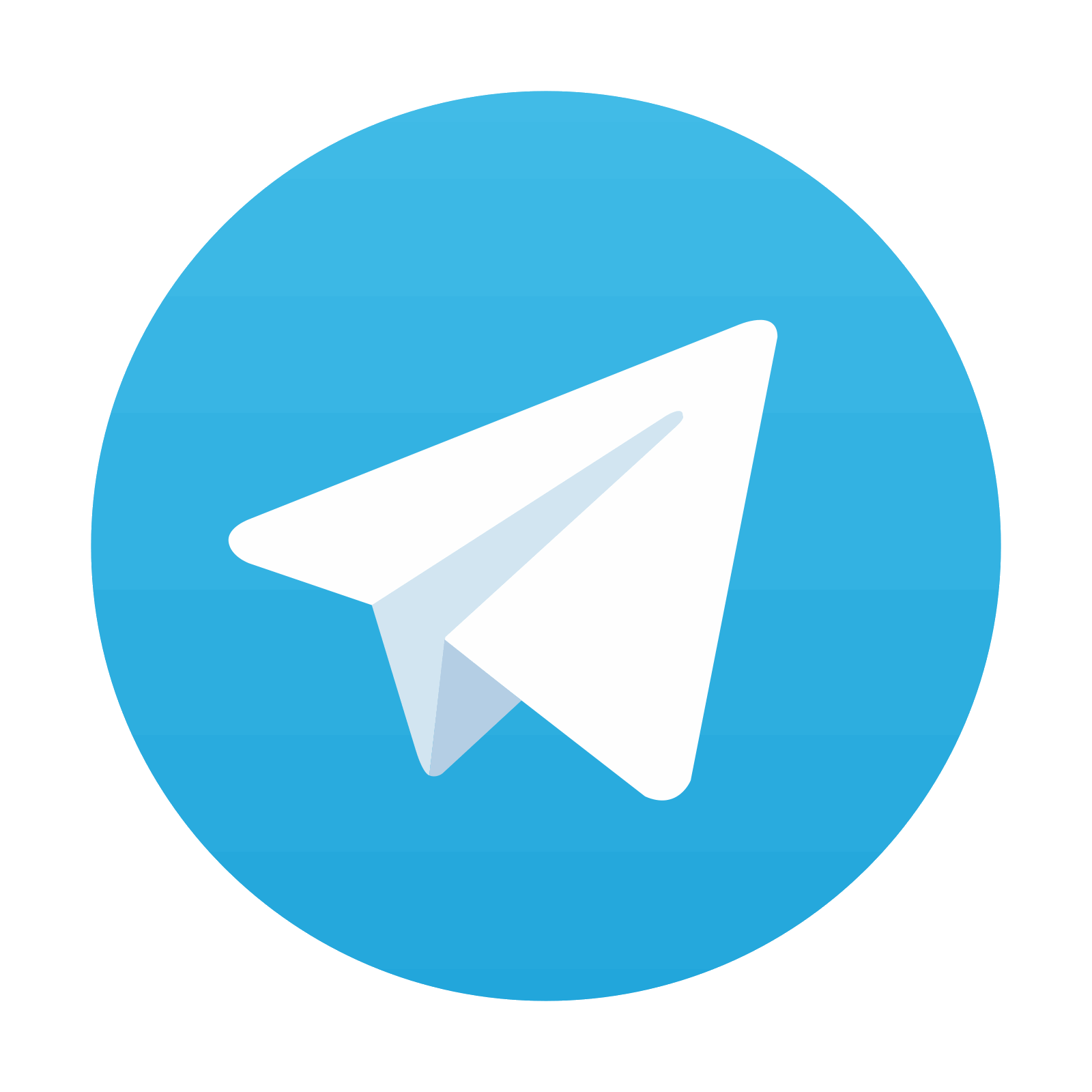
Stay updated, free articles. Join our Telegram channel
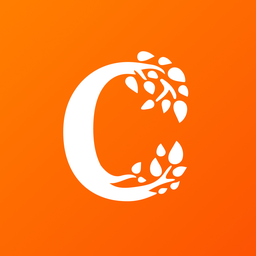
Full access? Get Clinical Tree
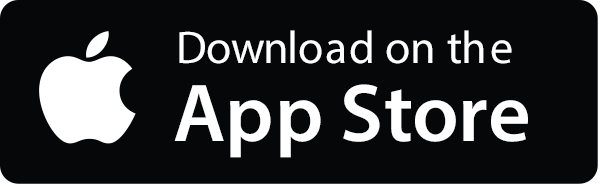
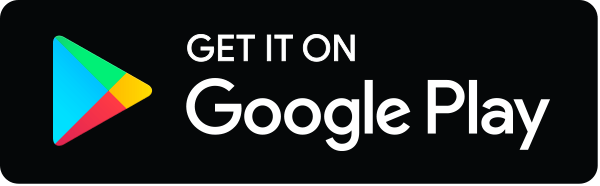