Fig. 9.1
In vivo axial spin-echo MRE magnitude and phase-difference data obtained from an anesthetized pig before (a, c) and immediately following (b, d) expiration. The green arrow shows the shear wave within the lung, emanating from the anterior chest wall and can be clearly identified when cardiac motion is absent (d). In the presence of cardiac motion, severe ghosting corrupts the phase-difference image (c)
Shear Wave Driver Technology
The thoracic cavity, rib cage, and pleural space pose unique challenges to the generation of uniform plane shear waves within the lung. Successful translation of MRE from the research environment into routine clinical practice will most likely be achieved through the use of so-called passive drum drivers in which the vibration of a flexible membrane on the surface of the driver in contact with the skin of the patient produces the necessary shear wave by means of mode conversion of longitudinal waves. Ideally, as little as possible tissue should separate the organ under interrogation and the driver membrane. Additionally, the tissue should be able to act as an effective couple between the driver and organ. The presence of the thoracic cavity, defined by the boney rib cage and intercostal muscles represents an effective rigid structure that serve to absorb a significant amount of energy transmitted from the driver face. The presence of the pleural space filled with pleural fluid further attenuates vibrations. A considerable effort is currently underway to investigate novel methods of shear wave generation as well as driver designs to overcome these limitations. Despite these challenges, Fig. 9.1b demonstrates that, even with existing passive driver technologies, shear waves can be generated within the lung in vivo.
Conventional (1H) MRE Methods
The application of 1H imaging for lung MRE has several advantages when compared to those techniques that employ exogenous contrast agents (see proceeding section) including the availability of numerous MRE pulse sequences that require relatively minor modifications and the lack of additional specialized hardware beyond the MRE driver technology used for shear wave generation.
As described previously, the ultra short T2* of lung parenchyma imposes limitations on the type of imaging sequence as well as specific parameters chosen for lung MRE. Consequently, the majority of work published thus far has focused on the development of spin-echo based 1H MRE imaging techniques. Figure 9.2 shows the first in vivo 1H spin-echo MRE lung images acquired from a normal volunteer. The four phase offsets were acquired during separate breath holds of 32 s while each phase offset was acquired with the following imaging parameters: TR/TE = 250/17 ms, k x /k y = 128/128, and a receiver bandwidth of 64 kHz. Shear waves were generated from a passive driver located at the lateral chest wall at a frequency of 40 Hz and can be seen propagating away from the chest wall towards midline [12].
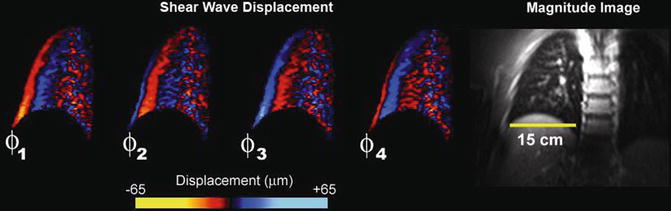
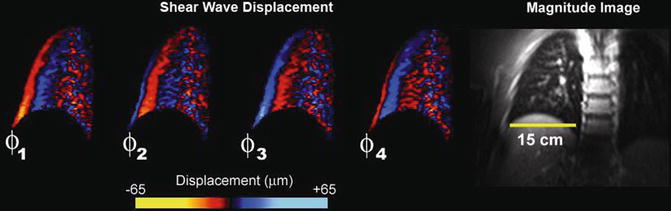
Fig. 9.2
Magnitude and phase-difference images acquired from a spin-echo MRE pulse sequence in a normal volunteer. The phase-difference images obtained at four equally spaced temporal offsets show the propagation of the shear wave from the lateral chest wall towards midline. Data reproduced from Goss et al. [12]
The choice of imaging parameters (i.e., TR and TE) for SE MRE applications is dictated by several tradeoffs. The choice of TR is governed by the balance between sufficient time for T1 signal recovery (choice of longer versus shorter TR) and sufficiently short imaging time to allow breath hold acquisitions (shorter versus longer TR). Similarly, the choice of TE is determined by the compromise between the need for a short as possible TE value to minimize intravoxel phase dispersion and signal loss and a longer TE in order to encode cyclic displacements at frequencies between the tens to hundreds of Hertz. In general, the choice of TR is based on the need to reduce overall imaging time. While the magnitude of the MR signal is affected by choice of TR, the phase of the signal, in which shear wave displacements are encoded is less so and as such shorter TRs are generally preferred. For both ex vivo, in vivo animal and human studies [12, 13], TRs of between 200 and 300 ms are typically used, making these imaging sequences highly T1-weighted. The overriding consideration for the choice of TE value is to make this parameter as short as possible. TEs can be minimized by choosing a driving frequency of the passive driver system to be several hundred Hertz as opposed to the typical value for the majority of abdominal MRE applications which is of the order of 40-60 Hz. With a driving frequency of 200 Hz, a TE of 22 ms can be achieved [13]. However, a disadvantage of the use of these relatively high frequencies is the decreased efficiency of generating longitudinal sound waves necessary to vibrate the passive driver as well as the increased attenuation of mode converted shear waves at these higher frequencies resulting from the viscous properties of tissue. The former problem can be partially overcome by increasing the driving voltage of the audio speaker used to generate the longitudinal sound waves. However, this poses increased performance requirements both in terms of the audio speaker as well as driving amplifier. In conventional spin-echo MRE pulse sequences, the interval between the 90° and 180° RF pulse is typically used to encode a single period of the shear wave motion. Thus, since shear frequency is inversely proportional to period, higher frequencies will reduce the period or time interval necessary to encode shear wave induced displacements and hence produce a lower TE.
To address the limitations of existing spin-echo MRE pulse sequences new, novel methods are currently being developed to both reduce the frequency of the longitudinal wave source while simultaneously reducing TE. These methods include reduction of the motion encoding gradient (MEG) duration and the incorporation of the MEGs into the crusher gradients which are typically played out on either side of the slice selection gradient of the 180° refocusing pulse. By incorporating the MEGs in this manner, the TE of the imaging sequence can be decreased significantly compared to the conventional spin-echo MRE pulse sequence. This is demonstrated in Fig. 9.3a, b which shows a conventional spin-echo based MRE pulse sequence with a bipolar 16.66 ms MEG pair with a TE of 44 ms and a modified spin-echo MRE pulse sequence with a TE of 10 ms respectively. The pulse sequence shown in 3a has the maximum sensitivity for 60 Hz shear motion. To achieve the pulse sequence design shown in 3b, the bipolar MEG pair was split into two 8.33 ms MEG lobes, each placed on either side of the 180° pulse. In this example the MEG lobes act as both motion encoding and crusher gradients.
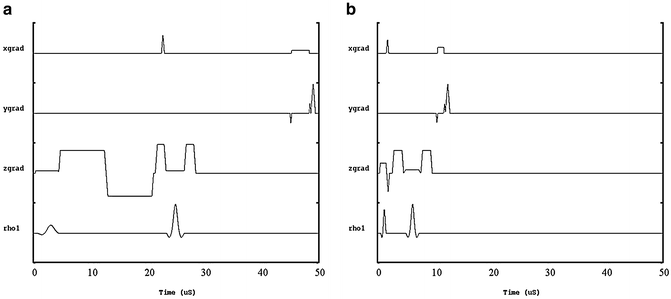
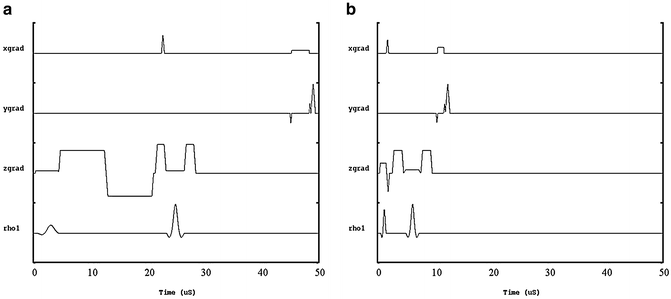
Fig. 9.3
(a) Conventional spin-echo based MRE pulse sequence with 16.66 ms bipolar MEG pair and a killer gradient on each side of the refocusing pulse, having a TE of 48 ms. (b) Modified MRE pulse sequence with two, 2 ms gradient lobes acting as MEG as well as a killer gradient having a TE of 10 ms
Hyperpolarized Gas Imaging of Lung Parenchyma
A novel method of addressing the limitations imposed by the ultra short T2* of lung parenchyma is to introduce an exogenous contrast agent in which the T2* of the agent is much longer than that of the surrounding parenchyma. Under these circumstances either gradient or spin-echo imaging sequences can be used for lung MRE. One such family of agents are Noble gas isotopes such as 3He and 129Xe which have been successfully employed to evaluate a variety of lung MR imaging applications including static and dynamic perfusion, diffusion [14], ventilation [15], and response to methacholine and exercise challenge [16]. Because these agents have an odd atomic mass number, they have a net magnetic moment that can be used as a contrast agent, similar to conventional 1H imaging. However, such agents typically have a relatively low net magnetic polarization under thermal (i.e., room temperature) conditions. The small signal resulting from the gas can be overcome by the process of hyperpolarization in which the polarization of the gas is increased from approximately 1 × 10−6 to approximately 10−1 [17]. Hyperpolarization is complex and expensive and as such, the concept of a centralized polarization facility has been developed to distribute the gas to sites that do not possess the necessary polarization hardware [17].
While hyperpolarized Noble gases can be used for both gradient and spin-echo imaging, the majority of applications have involved ultra fast gradient-echo imaging sequences using very small flip angles (<10°). This is because the magnetization achieved by means of hyperpolarization is non-renewable. The use of low flip angle gradient-echo pulse sequences ensures that sufficient magnetization will be available throughout the acquisition of all image echoes necessary for image formation. The use of ultra fast gradient-echo sequences has an additional advantage in that imaging can be performed on the order of several seconds, well within the breath hold time for some of the most ambulatory patients.
Figure 9.4 shows an example of performing MRE using a gradient-echo based MRE pulse sequence using hyperpolarized 3He in an ex vivo porcine lung. In this instance the imaging parameters were as follows; TR/TE = 100/8.3 ms, k x /k y = 128/64, bandwidth = 32 kHz, and field of view of 20 cm [13]. The presence of a shear wave emanating from the driver plate, as seen in the phase-difference images demonstrates that diffusion of the hyperpolarized gas within the respiratory zone of the lung is sufficiently restrictive so as to allow coherent phase accumulation of the magnetization of the gas and thus encode the displacement of the shear wave as it propagates throughout the lung. This suggests that hyperpolarized Noble gases have the potential for use as a contrast agent for lung MRE and that, while gases do not support shear, shear wave displacements can be encoded into the magnetization of a hyperpolarized gas because of gas trapping within the respiratory zone of the lung. The reconstructed shear wave map or elastogram also demonstrates regional changes in shear modulus throughout the lung, most likely due to the relatively higher pressure of the regions of the lung about the bronchi present within the imaging slice, from which the gas is perfusing from.
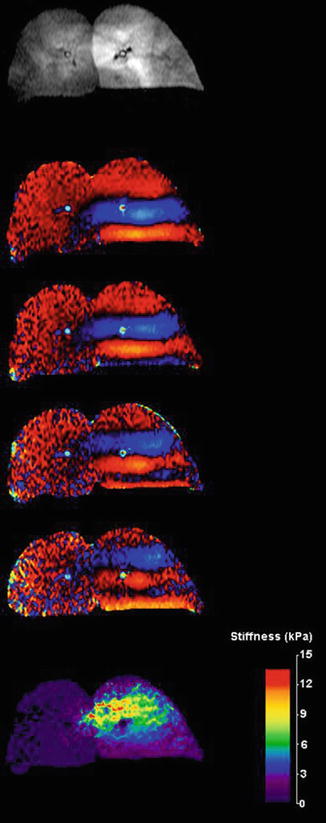
Get Clinical Tree app for offline access
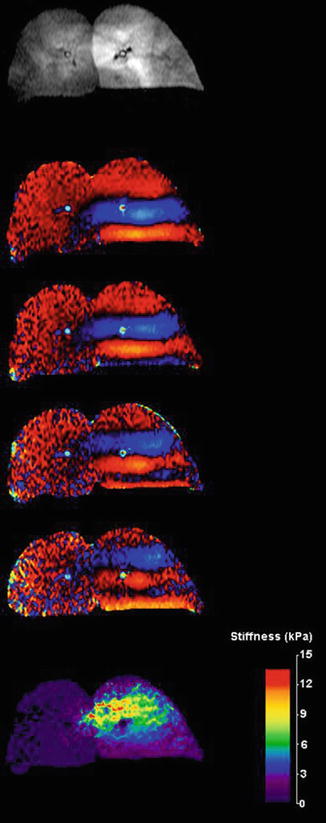
Fig. 9.4
Magnitude, phase difference, and reconstructed elastogram of an ex vivo porcine lung infused with hyperpolarized 3
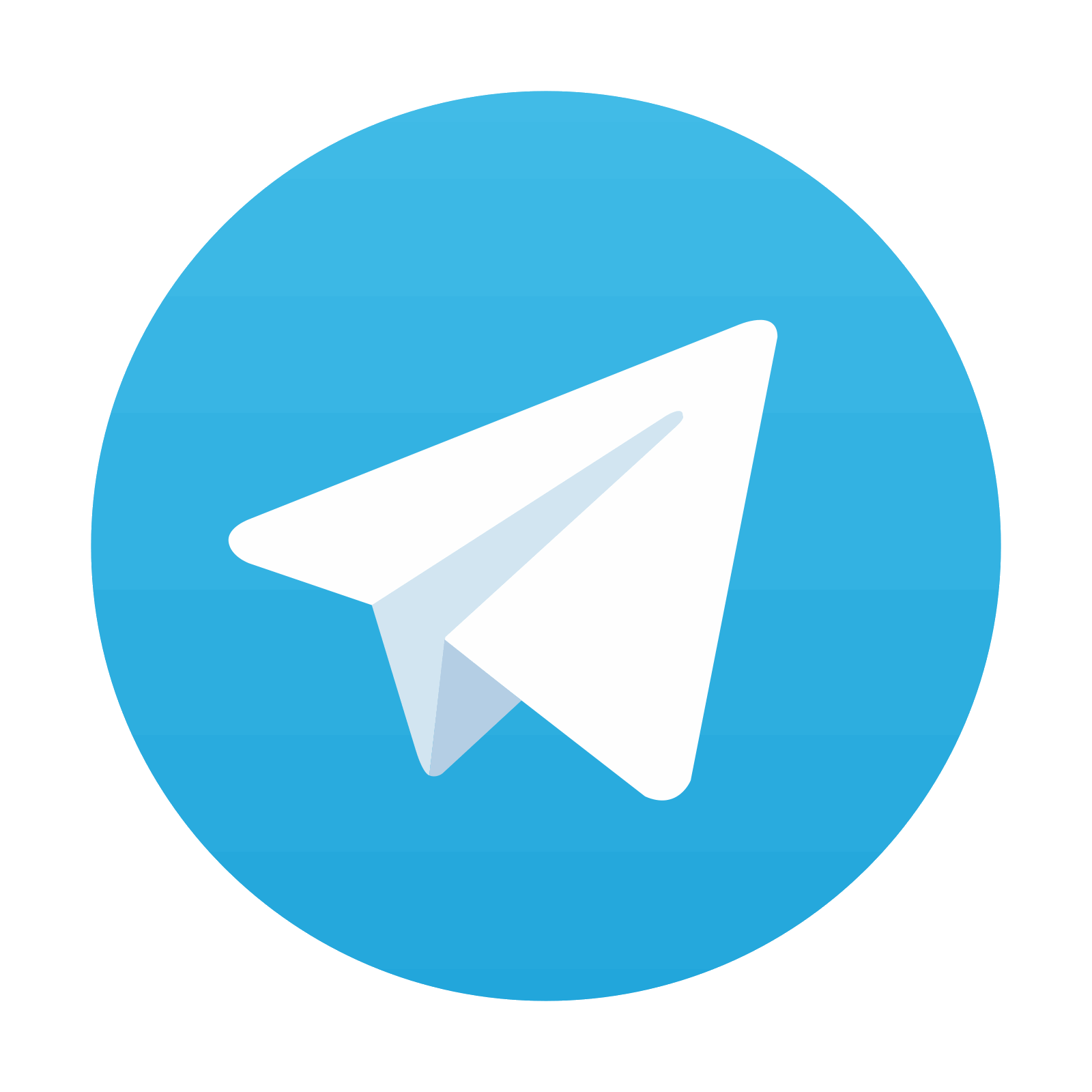
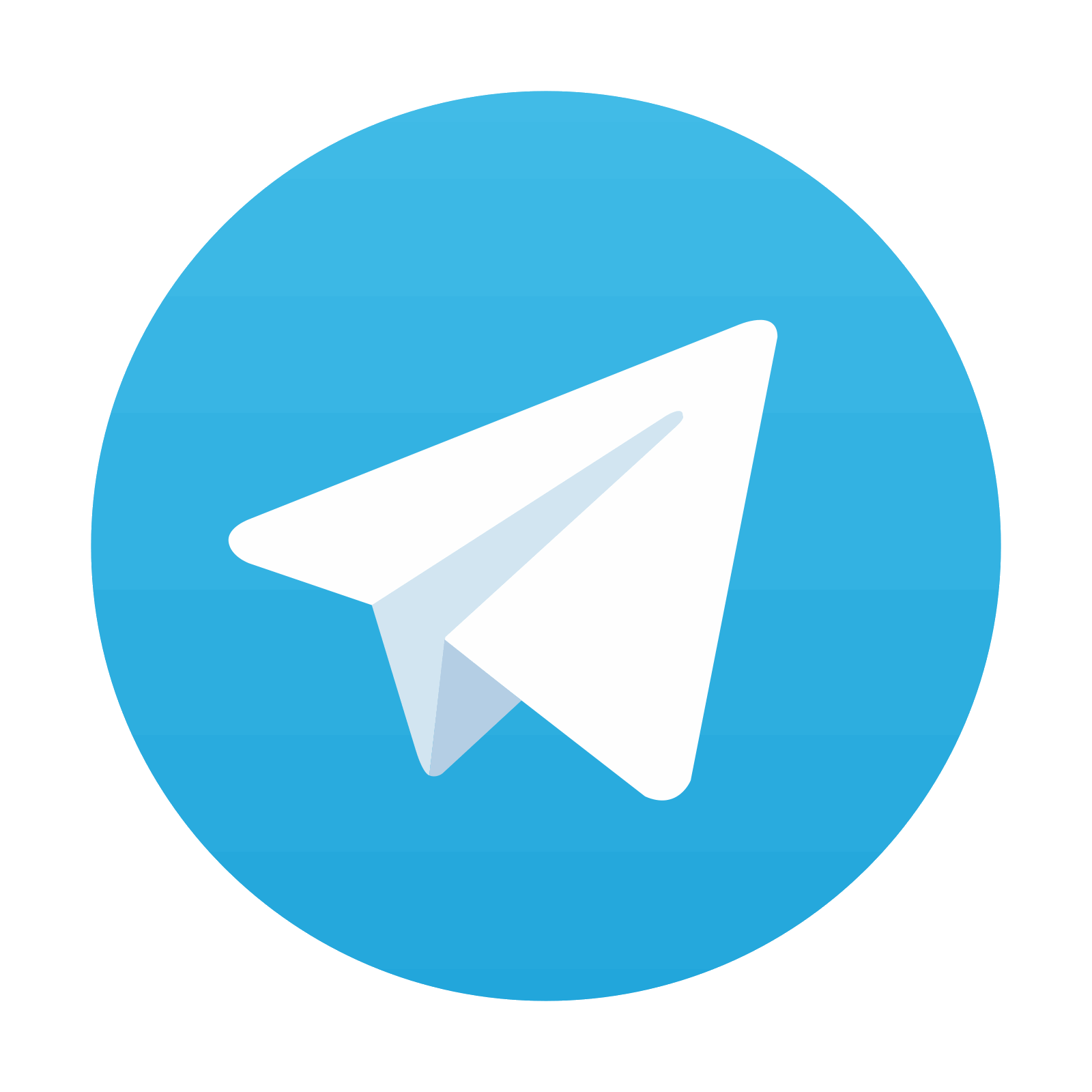
Stay updated, free articles. Join our Telegram channel
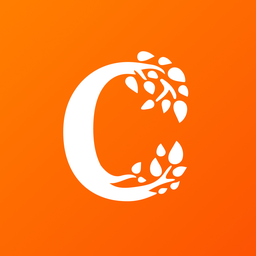
Full access? Get Clinical Tree
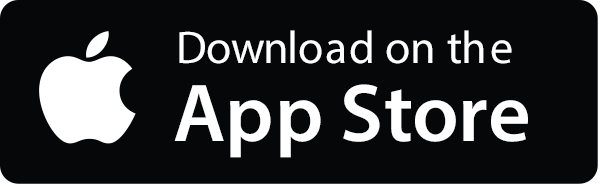
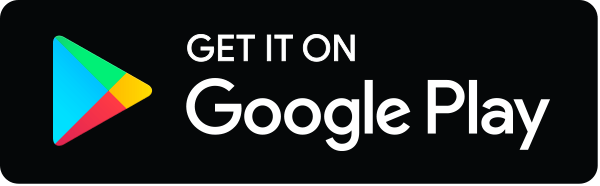
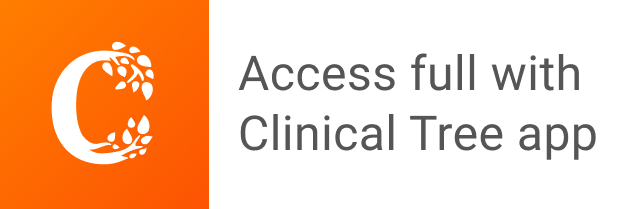