Fig. 1
Illustration of the 90Y microsphere radioembolization showing microcatheter in the hepatic artery releasing radioactive microspheres in the dominant flow leading to a tumor
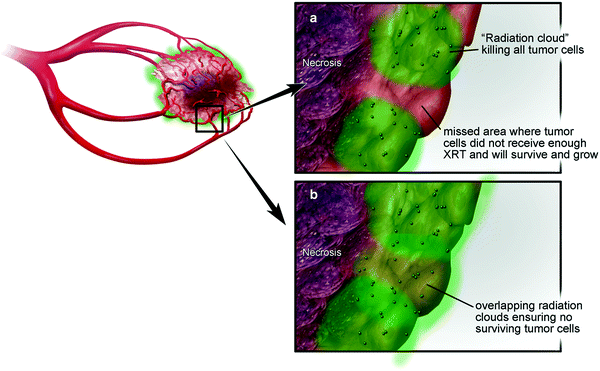
Fig. 2
Two possible scenarios depending on multiple factors: a incomplete coverage of radioactive fields and b intended implantation of adequate numbers of microspheres in sufficiently close proximity to each other
3 Historical Background of Radioactive Microparticle Therapy for Liver Cancers
The delivery of radioactive isotopes via vascular-borne microparticles to internal tumors dates back to 1947 when Muller and Rossier infused 63Zn and 198Au to treat renal cell metastases to the lungs, previously irradiated for bronchial carcinoma. Radioactive carbon particles that were used were 40–50 μm in diameter and were successfully trapped in bronchial tumors (Muller and Rossier 1947, 1951). Di Matteo reported the most extensive experience using 198Au particles delivered by intra-arterial injection to treat lung carcinoma, but subsequently abandoned this approach in favor of microsphere therapy (Di Matteo et al. 1962). Although most clinical experience is with 90Y, a variety of solid tumors have been treated with intra-arterial 32P particles suspended in solution as the therapeutic isotope (Dogliotti et al. 1966; Caldarola et al. 1965, 1966).
4 Physics of Radiation Therapy
4.1 Radiation Types
Radiation energy that causes ionization in the cell is of two types: electromagnetic or particulate. Electromagnetic energy, photons can be produced naturally by decay of radioactive isotopes (gamma rays) or by an electrical device accelerating electrons, which abruptly stop in a target, releasing energy (X-rays). The type of particulate energy most commonly used against malignant cells are electrons (charge −1, mass = 0.511 MeV), but others in limited use via external beam accelerators include protons (charge +1, mass = 2000 × electrons), alpha particles (helium ions), and neutrons (same mass as proton, no charge). In general terms, alpha particles are effective penetrating only up to 1 mm thick of tissue, beta is effective up to 3 mm, with gamma and neutron radiation passing completely through the body and stopping only in thick walls of concrete. Photons are discrete packets of electromagnetic energy which can cause cell damage via collision with a cell, transferring some of its energy to the cell. The exchange of energy to the cell deflects the path of the photon, with a resulting reduction in its energy. Energy absorbed by the cell can create damage to the DNA/RNA leading to cell death. A photons-only path of travel is linear and cannot be altered in the liver except by collision with tissue, and therein lies the key disadvantage in using photons (external beam radiation) for hepatic tumors. Normal tissues surround metastatic and primary cancers in the liver and are always either above, below, or beside the target tumor and will be in the entrance or exit path of the photon beam. Linear accelerators can produce electron beams, which differ from photon beams, in that electron are particles with mass and charge, and thus have a finite range of tissue penetrance, allowing for treatment of more superficial tumors, while significantly sparing deeper normal tissues. Electron beam therapy may be appropriate in treating a mass in the liver, which is only 1–2 cm deep to the surface. The dose 4 cm below the tumor could be nearly zero if the appropriate energy was chosen, compared to a dose of 80 % of the tumor dose at that depth, if photons were used. Protons can be used similarly to electrons, but with a much deeper penetration if required (Table 1).
Table 1
Radiation dose definitions
Radiation dose delivered | Effect/Result/Value |
---|---|
1 Gray (Gy) SI unit of dose | 1 Joule of energy deposited into 1 kg of tissue (absorbed dose) |
1 Gy Common Nomenclature | 100 cGy, (100 “rads” in older terms) |
1 Gy Striking Nucleus | 40—DNA double strand breaks (usually lethal to the cell) 1,000—DNA single strand breaks (often lethal to the cell) 4,000—DNA base modifications (possibly lethal to the cell) |
1 Gy External Beam Radiation | >300 cGy per min dose rate |
1 Gy High Dose Rate Gamma Brachytherapy (192Ir) | >20 cGy per min dose rate (typically 1 cm from source) |
1 Gy Low Dose Rate Brachytherapy (137Cs) | ~1 cGy per min dose rate at calculated point of interest |
1 Gy 90Y-microspheres | ~1 cGy per min inside 1 kg of mass with 1 GBq evenly distributed |
1 Gy 90Y-monoclonal Antibodies | 0.01 cGy sec−1 (systemic therapy for CD20+ lymphoma) Delivery method i.e., microsphere versus antibody will not affect dose rate which is GBq/kg |
4.2 Radiation Dose
Dose of ionizing radiation absorbed by the liver, solid tumor, or other tissues is a cornerstone of clinical trial design. Older reports used the term roentgen (R), which described ionization in air, i.e., exposure of gamma rays. Newer nomenclature uses the SI unit for absorbed dose in tissue (1 Joule/kg = 1 gray (Gy) = 100 rads = 100 cGy (centigray)), as the basic unit of measurement. Conversion of older literature values listed as R is approximately 1 R = 0.01 Gy for gamma. It is less well known how to convert beta radiation doses, which are low dose, constant release radiotherapy, into equivalent external beam doses due to the differences in biologic response due to dose rate, fractionation, and activity (Zeman 2000). Thus brachytherapy doses are recorded as Gy, but these doses are not likely to be equivalent to the same dose Gy given as daily fractionated external beam doses of X-rays. This is an area of active investigation.
4.3 Brachytherapy
It was not long after Dr. Wilhelm Conrad Roentgen discovered X-rays in 1895 that the Lancet reported its use in January 1896 for medical use (Hall 2000). Shortly after the turn of the century, it was suggested by Alexander Graham Bell that radioactive isotopes be applied directly to tissues, and thus brachytherapy was born—from the Greek “brachy” meaning “short range”. The French coined the term endocurietherapy, Greek “endo”, meaning “within”. Radioactive isotopes such as iridium (192Ir), cesium (137Cs), and iodine (125I and 131I) have been used extensively since the early 1900s as primary therapy, and in addition to external beam radiation as a ‘boost’ to the tumor. Brachytherapy attempts to spare normal regional tissues by delivering a high dose locally in the tumor, and although gamma radiation photons are used mostly, there is relatively low dose at a distance from the tumor of several centimeters. The dose rate of radiation delivery via a brachytherapy isotope (50 cGy/h) is much lower than photons delivered by an accelerator, (500–2,400 Gy/min). Radioactive decay from an isotope that produces electrons (charge −1) is termed “beta decay”. These particles are used in such products as radiolabeled antibodies used in hematologic malignancies, or in higher energies, for bone metastases and thyroid malignancies. Currently, there is significant clinical use of pure beta emitting isotopes (no gamma photons emitted) yttrium and strontium (90Y, 90Sr) in brachytherapy in liver lesions and systemically with antibody carriers (Wiseman and Witzig 2005; Wiseman et al. 2003; Knox et al. 1996; Macklis et al. 1994). An advantage and potential disadvantage of beta sources is that most of the effective radiation is delivered within 2–4 mm of the source, with virtually no radiation dose effect >1 cm away. Because there are no gamma rays, nuclear medicine detectors cannot readily image pure beta sources, making localization of implanted sources problematic. Brachytherapy sources can be implanted via blood infusion, needle applicator, directly applied and sutured into place as a permanent implant, or placed temporarily (minutes–hours) within a catheter that is removed from the body.
5 Radiobiology
An understanding of radiation effects in living tissues began at the turn of the century with observations of skin reaction, primarily erythema and breakdown (Hall 2000). Since then clinical experience has produced observations regarding normal and malignant tissue response and repair to ionizing radiation. DNA must be damaged and remain unrepaired or misrepaired to cause loss of reproductive ability or initiate apoptotic death. It has been estimated that in the presence of sufficient oxygen tension (>10 mm Hg) (Hall 2000; Kennedy et al. 1997) any form of radiation (X-rays, gamma rays, charged or uncharged particles) will be absorbed and potentially interact with the DNA. Approximately 75 % of the damage to the DNA is indirect, with a photon striking a water molecule (water is 80 % of the cell) within 4 nm of the DNA strand. Kinetic energy from the incident photon is transferred to an orbital electron of the water molecule, ejecting it, now called a secondary electron. Energy transferred to a water molecule forms a free radical, which is highly reactive and breaks bonds of DNA strands nearby. There can also be interaction of the secondary electron directly on the DNA strand causing damage, referred to as direct action (Hall 2000).
5.1 Modifiers of Radiation Response
The presence of oxygen is the single most important biologic modifier of radiation effect at the cellular level (Zeman 2000; Withers 2002). Oxygen is needed to make radiation damage caused by free radicals “permanent”, however, in a hypoxic state this damage can be repaired. The ratio of radiation dose without oxygen compared to the dose with oxygen which will produce the same biologic effect is termed the “oxygen enhancement ratio” or OER. For X-rays OER is between 2 and 3, i.e., a given X-ray will be 2–3 times as damaging in the presence of oxygen to the cell than it will in a hypoxic milieu (Hall 2000). This has significant implications clinically for microsphere therapy as many patients with liver malignancies are first considered for embolization procedures (TACE and Bland Embolization), which will likely produce a relative hypoxic environment within the tumor mass and reducing the OER. Other factors known to impact radiation effectiveness are widely known as the 4 “R”s: Reoxygenation (OER); Repair of radiation damage, Reassortment of cells into more or less sensitive portions of the cell cycle (S phase most radioresistant, G2-M most sensitive); Repopulation during a course of radiation, which is seen in rapidly dividing tumor populations, however, the continuous low dose of radiation over 14 days delivered by 90Y can overcome this possible factor. Repopulation can also become an issue after surgical resection, chemoembolization, cryotherapy, or radiofrequency ablation, where hepatic hypertrophy in the regional normal cells is stimulated. These normal clonogens are more susceptible to radiotherapy damage in this phase, limiting the use of radiation, which may allow for residual malignant cells to repopulate (Lawrence et al. 1995). Repair of radiation damage or “sublethal damage repair” is enhanced in low oxygen environments and with fractionation (multiple radiation doses). The break (24 h typically) between each fraction of external beam radiotherapy provides opportunity to repair DNA strand breaks in normal and malignant cells. Brachytherapy differs in this regard with continuous radiation, without a discrete “fraction” of radiation, but delivers lower dose rate of radiation continually.
6 Radiation Effects in the Liver
Acute and late effects of ionizing radiation to the liver have been described in the literature since the early 1960s (Ingold et al. 1965; Ogata et al. 1963). During radiotherapy, acute transient effects are often reflected as elevation of liver transaminases, and depending upon the treated volume, hematologic effects such as neutropenia and coagulopathy can occur. However, permanent effects can be produced, occurring weeks or months after radiation (“late effects”) such as fibrosis, persistent enzyme elevation, ascites, jaundice, and rarely, radiation-induced liver disease (RILD) and fatal veno-occlusive disease (VOD) (Lawrence et al. 1995, 1992; Austin-Seymour et al. 1986; Dawson et al. 2001). RILD is often what is called “radiation hepatitis” and classically was described as occurring within 3 months of initiation of radiation, with rapid weight gain, increase in abdominal girth, liver enlargement, and occasionally, ascites or jaundice, with elevation in serum alkaline phosphatase. The clinical picture resembled Budd-Chiari syndrome, but most patients survived, although some died of this condition without proven tumor progression. It was described that the whole liver could not be treated with radiation above 30–35 Gy in conventional fractionation (1.8–2 Gy/day, 5 days per week) or else RILD or VOD was likely to occur. Interestingly, VOD can also occur without radiotherapy in patients receiving high dose chemotherapy in hematologic malignancies, alkaloids, toxic exposure to urethane, arsphenamine, and long-term oral contraceptives, (Fajardo et al. 2001) as well as patients receiving radiation combined with chemotherapy or radiation alone. The clinical presentation can differ between RILD and chemotherapy plus radiation liver disease, but the common pathological lesion associated with RILD is VOD. The pathologic changes in VOD can affect a fraction of a lobe, or the entire liver. It is best observed on low power microscopy, which demonstrates severe congestion of the sinusoids in the central portion of the lobules with atrophy of the inner portion of the liver plates (zone 3) (Lawrence et al. 1995; Fajardo et al. 2001). Foci of yellow necrosis may appear in the center of affected areas. If the affected area is large, it can produce shrinkage and a wrinkled granular capsule. The sublobular veins show significant obstruction by fine collagen fibers, which do not form in the larger veins and (suprahepatic and cava) which is a distinction between RILD and Budd–Chiari syndrome (Lawrence et al. 1995; Fajardo et al. 2001). Most livers heal and will display chronic changes after 6 months with little congestion, but distorted lobular architecture with variable distances between central veins and portal areas. These chronic liver changes are typically asymptomatic but are reproducibly seen on liver biopsies as late as 6 years after presentation. Further investigation of the pathogenesis of VOD is difficult as most animals do not develop VOD in response to radiation (Fajardo et al. 2001). Unfortunately, no animal model exists to study VOD. Hahn used radioactive colloid of up to 67,000 cGy of 198Au in dogs that survived up to 62 days (Hahn et al. 1951). Wollner demonstrated that dogs treated with glass 90Y microspheres dosed up to 35,480 cGy survived without developing VOD or liver failure. This dosage far exceeds the level of liver radiation humans could tolerate (Wollner et al. 1988). Extensive radiation damage was noted, including necrosis and fibrosis mainly in the central vein regions, and numerous microspheres that had congregated in the gallbladder wall. Long-term survivors retained a multinodular, firm, and shrunken liver compared to dogs receiving nonradioactive microspheres (Wollner et al. 1988). Similar results were noted by Wollner in dogs with hepatic artery infusion of 5-bromo-2′-deoxyuridine (BUDR) concurrent with delivery of resin or glass 90Y microspheres (Wollner et al. 1987) (Fig. 3).