Fig. 14.1
This diagram depicts the typical schematic of a bifunctional molecular imaging agent. The imaging moiety (signal generation molecule) on the left connects to a targeting moiety on the right. The imaging moiety permits signal detection by a range of modalities including PET, SPECT, MRI, ultrasound, or optical imaging. The targeting moiety provides the “zip code” for delivering the agent to its intended molecular or cellular target. The imaging moiety might consist of a peptide or small molecule, a nanoparticle that can house multiple targeting moieties, and/or a therapeutic payload. The latter class of bifunctional agents, known as theranostic agents, combines a diagnostic imaging agent with targeted delivery of a therapeutic agent (Reprinted from Libby P, Jaffer FA, Weissleder R. Molecular imaging in cardiovascular disease. In: Bonow RO, Mann DL, Zipes DP, Libby P (eds). Braunwald’s Heart Disease: A Textbook of Cardiovascular Medicine, 9th edition. Philadelphia, PA: Elsevier Saunders; 2011: 448–458. With permission from Elsevier)
Imaging moieties can permit visualization by a number of different modalities that each offer strengths and weaknesses (Table 14.1). These modalities include isotopic approaches that offer great sensitivity or signal-to-background ratio, but generally lack precise localization. Nuclear imaging approaches have the advantage of ready availability, and in the case of clinically used isotopic agents, considerable tissue penetration. Microbubbles used for contrast-enhanced ultrasound, coupled with antibodies or other ligands that serve as targeting moieties, also benefit from a large installed base of ultrasound equipment. Magnetic resonance imaging (MRI) methods that use paramagnetic imaging agents provide favorable contrast-to-noise ratios and good anatomical localization, but have limitations in application to the coronary arteries due to respiratory and cardiac motion during the time span required for optimum image acquisition (see Chap. 13). Optical approaches, such as near-infrared fluorescent (NIRF) technologies, have undergone considerable experimental development for molecular imaging of atherosclerosis. For human coronary artery imaging, however, tissue penetration severely limits external optical imaging. Thus, for coronary arterial applications, NIRF techniques will require intra-arterial approaches, as discussed below. Table 14.1 summarizes the strengths and weaknesses of some of the modalities that could serve in molecular imaging of the human coronary arterial tree.
Table 14.1
Overview of molecular imaging modalities
Technique | Resolution | Depth | Time | Quantitation | Multichannel imaging | Imaging agents | Target | Cost | Primary small animal use | Clinical use |
---|---|---|---|---|---|---|---|---|---|---|
MR imaging | 10–100 μm | No limit | Minutes to hours | Absolute | Multiple | Paramagnetic chelates, magnetic particles | A, P, M | $$$ | Versatile imaging modality with high soft tissue contrast | Yes |
CT imaging | 50 μm | No limit | Minutes | Absolute | Multiple | Iodine | A, P, Ma | $$ | Primarily for vascular, lung, and bone imaging | Yes |
Ultrasound imaging | 50 μm | Centimeters | Seconds to minutes | Absolute | Multiple | Microbubbles | A, P, Ma | $$ | Vascular and interventional imaging | Yes |
PET imaging | 1–2 mm | No limit | Minutes to hours | Absolute | No | 18F-, 64Cu-, 11C-, and 68Ga- labeled compounds | P, M | $$$ | Versatile imaging modality with many different tracers | Yes |
SPECT imaging | 1–2 mm | No limit | Minutes to hours | Absolute | Two | 99mTc-, 111In-, 131I-labeled compounds; 67Ga, 201Tl | P, M | $$ | Commonly used to image labeled antibodies, peptides, or perfusion | Yes |
Fluorescence reflectance imaging | 1 mm | <1 cm | Seconds to minutes | Relative | Multiple | Photoproteins, fluorochromes | P, M | $ | Rapid screening of molecular events in surface-based disease | Yes |
Fluorescence-mediated tomography | 1 mm | <10 cm | Minutes | Absolute | Multiple | Near-infrared fluorochromes | P, M | $$ | Quantitative imaging of targeted or “smart” fluorochrome reporters | In development |
Bioluminescence imaging | Several millimeters–centimeters | Centimeters | Minutes | Relative | Multiple | Luciferins, coelenterazines, luminol | M | $$ | Gene expression, cell and bacterial tracking, protein processing, and MPO activity | Potentially in development |
Intravital microscopy (e.g., confocal, multiphoton) | 1 μm | <400–800 μm | Seconds to hours | Relative | Multiple | Photoproteins, fluorochromes | A, P, M | $$$ | All of the above at higher resolutions but at limited depths and coverage | In development (endoscopy, skin) |
Targets for Molecular Imaging of Coronary Atherosclerosis
Biological investigations of the pathophysiology of atherosclerosis have revealed several potential targets for molecular imaging (Fig. 14.2). The particular biological processes discussed below comprise a partial listing of these processes. The targets detailed here, while not comprehensive, represent those for which exploitation for molecular imaging of atherosclerosis has progressed the most, and that promise the greatest ease of translation to clinical use.
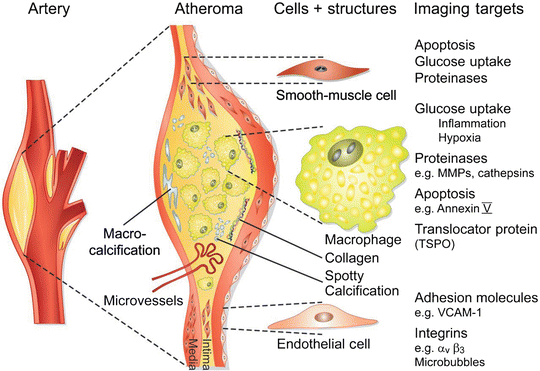
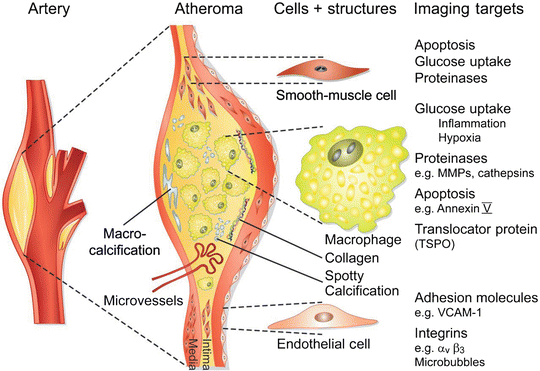
Fig. 14.2
Molecular imaging targets in atherosclerotic plaque. The left diagram shows a plaque in the carotid bifurcation. The magnified view of the plaque in the middle shows its composition, including a calcified region in light blue at the base of the plaque on the left, macrophages (yellow) in the lipid-rich necrotic core, triple helical collagen fibrils, and smooth-muscle cells within the fibrous cap (brown) below the endothelial monolayer. Spotty calcification is shown as blue dots. Microvessels may penetrate the plaque’s base, starting from the adventitia. Key imaging targets on the major cell types or in the plaque matrix shown are listed on the right. MMPs matrix metalloproteinases, VCAM-1 vascular cell adhesion molecule-1 (Reprinted from Camici PG, Rimoldi OE, Gaemperli O, Libby P. Non-invasive anatomic and functional imaging of vascular inflammation and unstable plaque. Eur Heart J 2012;33:1309–1317. With permission from Oxford University Press)
Endothelial Cell Activation
The explosion of understanding of endothelial cell functions during atherogenesis has provided some potential targets for molecular imaging. When the endothelial cells encounter signals implicated in atherogenesis, they augment the expression of several structures, from the usually at low levels in the basal state. Resting endothelial cells, for example, resist prolonged contact with blood leukocytes. When exposed to pro-inflammatory stimuli implicated in atherogenesis—notably, certain cytokines—endothelial cells display on their surface adhesion molecules that selectively bind various classes of leukocytes. As an iconic example, endothelial cells in normal human arteries have low levels of an adhesion molecule known as vascular cell adhesion molecule-1 (VCAM-1), which binds just those types of leukocytes that accumulate in atherosclerotic plaques—most prominently, monocytes/ macrophages and T lymphocytes [5]. In animals that consume an atherogenic diet, or in vitro when stimulated with pro-inflammatory cytokines, endothelial cells express high levels of VCAM-1 [6]. Some approaches to targeting VCAM-1 also have served to target molecular imaging agents—including antibodies bound to microbubbles for contrast-enhanced ultrasonography, and various generations of peptides linked to near-infrared, isotopic, or MRI contrast moieties. Multimodality agents directed at VCAM-1 have successfully imaged atheromata in the aortic root of mice (Fig. 14.3).
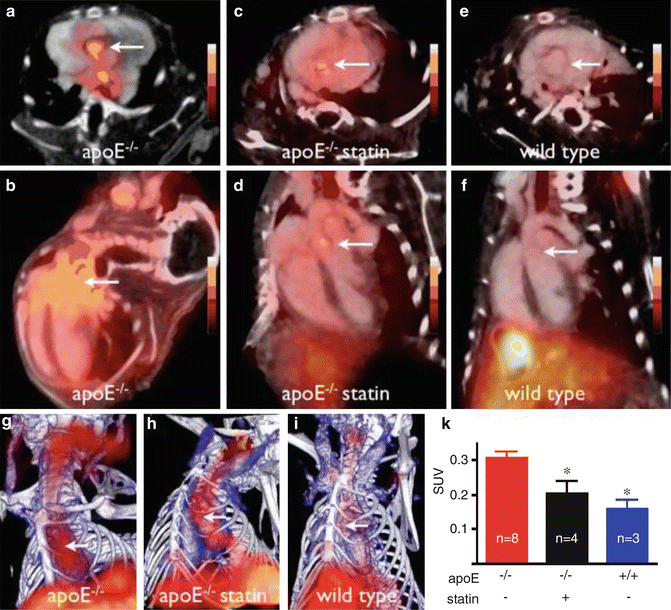
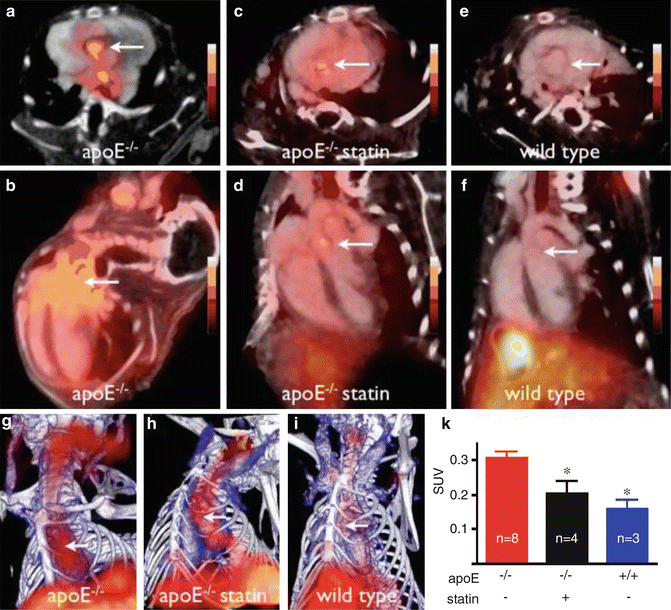
Fig. 14.3
Integrated PET/CT imaging of VCAM-1 expression in apoE−/− mice without and with statin treatment. A tetrameric affinity peptide with high affinity and specificity for VCAM-1 was derivatized with 1,4,7,10-tetraazacyclododecane-1,4,7,10-tetraacetic acid (DOTA) and then 18F labeled and tested in apoE−/− mice, without or with statin treatment. PET detects VCAM-1 expression, and CT provides co-registered anatomical information. (a, c, e) PET-CT short-axis images of the aortic root of apoE−/−or wild-type mice (arrows). (b, d, f) Long-axis views. (g, h, i) Maximum intensity projection with 3D representation. Bone = white; vasculature = blue; 18F-4 V = red. K: Standard uptake value (SUV) graph of VCAM-1 distribution across the three different groups, *p < 0.05 (Reprinted from Nahrendorf M, Keliher E, Panizzi P, et al. 18F-4 V for PET-CT imaging of VCAM-1 expression in inflammatory atherosclerosis. JACC Cardiovasc Imaging 2009; 2 (10): 1213–1222. With permission from Elsevier)
Angiogenesis
Hypoxia in evolving atheroma stimulates plaque neovascularization, either from residing endothelial cells (angiogenesis) or from recruited endothelial progenitor cells (vasculogenesis) [7]. Plaque hypoxia accelerates foam cell stress and death, as well as growth and expansion of the necrotic core. While new vessel growth serves to counteract tissue hypoxia, the fragile neovasculature may readily rupture, leading to intraplaque hemorrhage and lesion expansion. Plaque neovessels may also support lesion growth through a nutritive function. Reduction of penetrating vasa vasorum in atheromata thus might reduce intraplaque hemorrhage and lesion growth. In any case, the presence of angiogenesis characterizes advanced, potentially high-risk atheroma and has evolved as a leading molecular imaging target.
Integrins
Integrins mediate many cell–cell and cell–matrix adhesion processes that occur during angiogenesis. In particular, neovessels express abundant integrin alpha-v beta-3 (αvβ3), a potentially useful in vivo molecular imaging target. Integrin αvβ3 notably binds the tripeptide RGD motif (arginine–glycine–aspartic acid).
Lanza, Wickline, and colleagues first demonstrated imaging integrin αvβ3 expression in atheroma in vivo via noninvasive MRI [8]. Utilizing a gadolinium-coated perfluorocarbon nanoscaffold (90,000 gadolinium chelates per scaffold) derivatized with an RGD peptidomimetic, the authors imaged plaque angiogenesis in hyperlipidemic New Zealand white rabbits. Compared to untargeted perfluorocarbon agents, the targeted agents doubled the segmented MRI signal enhancement. Other control groups, including normal rabbits injected with the targeted agent and atheroma-bearing rabbits pre-injected with an unlabeled targeted agent, demonstrated significantly less MRI signal enhancement. Immunostaining of neovessels by integrin αvβ3 demonstrated greater expression in atheroma compared to the normal vessel wall.
For near-term clinical translation of angiogenesis molecular imaging, the agent 18F-Galacto-RGD appears intriguing. It has undergone clinical testing in cancer patients [9], providing a precedent for extension to patients with coronary artery disease using integrated positron emission tomography/computed tomography (PET/CT). Of interest, an experimental study imaging mouse atheroma without angiogenesis demonstrated that 18F-Galacto-RGD might colocalize with plaque macrophages [10], and therefore might report on both angiogenic vessels and macrophages in human plaque.
Leukocyte Accumulation
Once attached to the endothelium via interactions with adhesion molecules such as VCAM-1, leukocytes enter the arterial intima. A subset of pro-inflammatory cytokines known as chemokines likely mediates much of this directed migration. Having taken up residence in the arterial plaque, these phagocytes provide a number of targets for molecular imaging. Monocytes that have penetrated into the intima mature into macrophages. They often accumulate lipids and form the foam cells characteristic of atheromatous lesions. Imaging approaches that depend on the phagocytic activity of macrophages are considered below. A subset of macrophages in the atherosclerotic plaque contains the enzyme myeloperoxidase (MPO). This enzyme generates the highly oxidant species, hypochlorous acid (HOCl). Various probes can visualize MPO activity and/or its product, hypochlorite [11, 12].
The mitochondrial membrane in mononuclear phagocytes contains a transporter molecule known as TSPO (translocator protein)—or previously, as the peripheral benzodiazepine receptor. Small molecule ligands that recognize this transporter molecule, coupled to isotopic agents detectable by PET, have served to image inflammation in large arteries in humans [13, 14]. This approach awaits application to smaller arteries, such as those in the coronary tree.
Phagocytic Capacity
Macrophages characteristically exhibit phagocytic activity, allowing them to engulf infectious agents, foreign bodies, and—in the context of atherosclerosis—lipids and various lipoproteins. Some approaches to molecular imaging of atherosclerosis have exploited the phagocytic property of macrophages. Ultrasmall superparamagnetic iron oxide (USPIO) particles can accumulate in macrophages and provide MRI contrast [15]. Experimental studies have demonstrated the feasibility of plaque imaging using this approach. Using suppression of T2-weighted signals, studies have quantitatively correlated macrophage content with such superparamagnetic microparticles in atheromata of a rabbit aorta, a vessel of similar caliber to human coronary arteries (Fig. 14.4) [16].
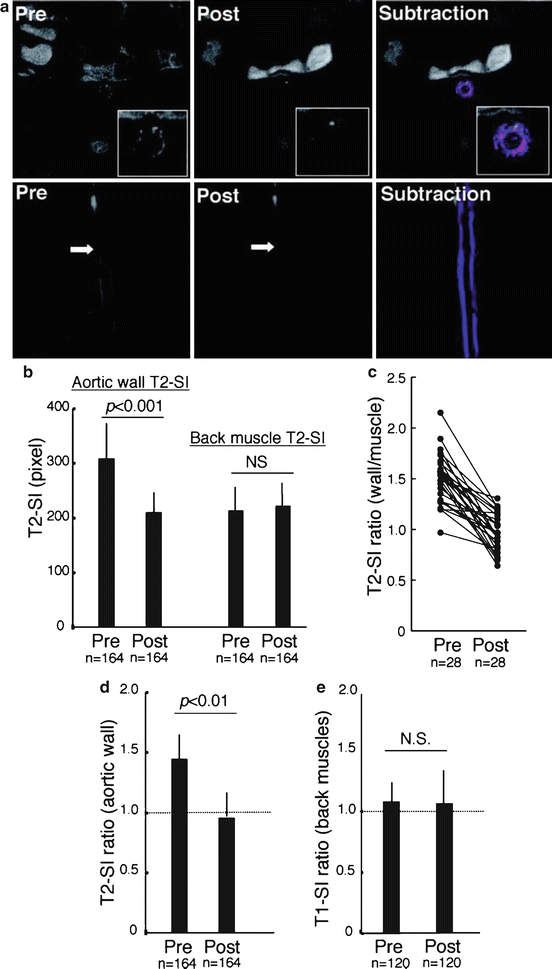
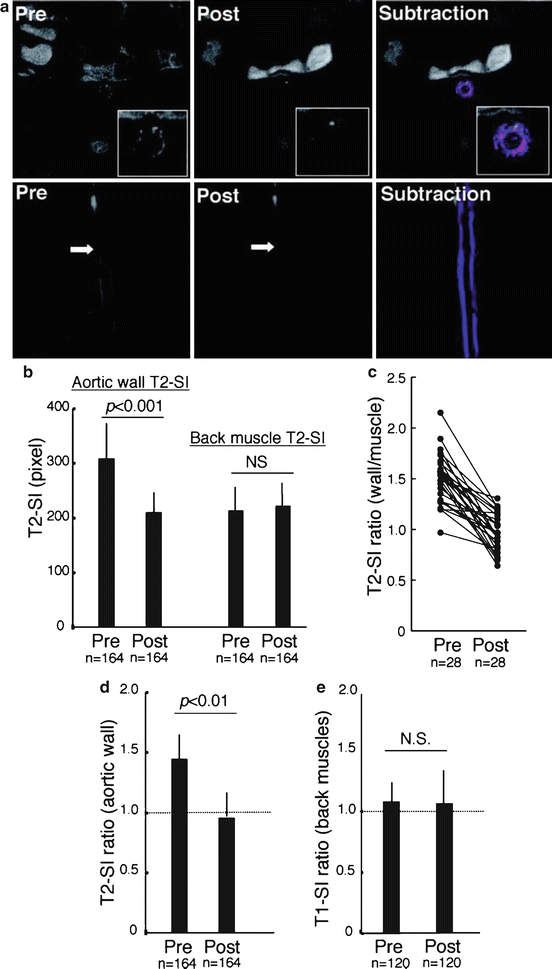
Fig. 14.4
Molecular MRI of plaque macrophages in rabbit aorta using superparamagnetic nanoparticles (MION-47). MION-47 is internalized within plaque macrophages. T2-weighted pulse sequences were used to visualize MION-47-induced MRI signal loss in vivo. (a, top row) Axial images (pre and post) 72 h after MION-47 injection, with subtraction image (top right, pseudocolored) further demonstrating the net effect on the MRI signal. (Bottom row) Coronal view of the same aorta showing diffuse MRI signal loss throughout the aortic wall, with varying magnitudes. Arrows indicate the cross section obtained for axial images in the top row. (b) MION-47 induced a significant reduction in the aortic T2-signal intensity (SI) (N = 164 aortic wall levels, p < 0.001). Of note, T2-SI in muscle was not changed by MION-47. (c) Average T2-SI ratio of the aortic wall (T2-SI divided by back muscle T2-SI) of a single representative rabbit, across all aortic sections. (d) MION-47 induced a significant reduction in the T2-SI ratio (p < 0.01, N = 6 rabbits, 164 cross sections), but not in the T1-SI ratio (120 slices, 6 rabbits), in the aortic wall (Reprinted from Morishige K, Kacher DF, Libby P, et al. High-resolution magnetic resonance imaging enhanced with superparamagnetic nanoparticles measures macrophage burden in atherosclerosis. Circulation 2010; 122 (17): 1707–1715. With permission from Wolter Kluwers Health)
Proteinase Activity
A plethora of findings, over the last two decades, support the participation of proteinases in arterial remodeling during atherogenesis and the disruption of atherosclerotic plaques [4]. In particular, degradation of interstitial collagen in the plaque’s fibrous cap likely renders it susceptible to rupture. Such disruptions of coronary atherosclerotic plaques precipitate a majority of fatal acute myocardial infarctions. The principal collagenolytic enzymes implicated in this process include members of the matrix metalloproteinase (MMP) family. We have shown overexpression of the three principal MMP interstitial collagenases in human atherosclerotic plaques (MMP-1, -8, and -13) [4]. Our extensive gain-of-function and loss-of-function data in atherosclerotic mice, demonstrated directly a role for MMP interstitial collagenases in the economy of collagen in mouse atherosclerotic plaques [4, 17]. Our group has also provided evidence for the involvement of certain potent elastases—including the cysteinyl proteinases cathepsins S, K, and L—in human and experimental atherosclerosis and in experimental and human aneurysmal disease [18].
Several experimental studies have demonstrated the feasibility of imaging these classes of proteinases. NIRF-emitting beacons that assess proteinase activity by liberation of quenched fluorescent moieties following proteolysis, pioneered by the laboratory of Dr. Ralph Weissleder, have proven pivotal in this regard. We visualized the interstitial collagenase MMP-13 in mouse atherosclerotic plaques using this approach [19]. Probes activated by cathepsin K also permit visualization of mouse atheromata [20]. Ex vivo studies of freshly excised human carotid atherosclerotic plaques provide evidence that supports the clinical translatability of such approaches to proteinase imaging. Other laboratories have used radionuclide-labeled proteinase inhibitors to visualize plaque proteolytic enzymes [21]. While the one-to-one stoichiometry of the inhibitor-based approaches does not utilize the catalytic amplification provided by enzymatic activity, the sensitivity and tissue penetration of nuclear approaches might permit external imaging of human atherosclerosis.
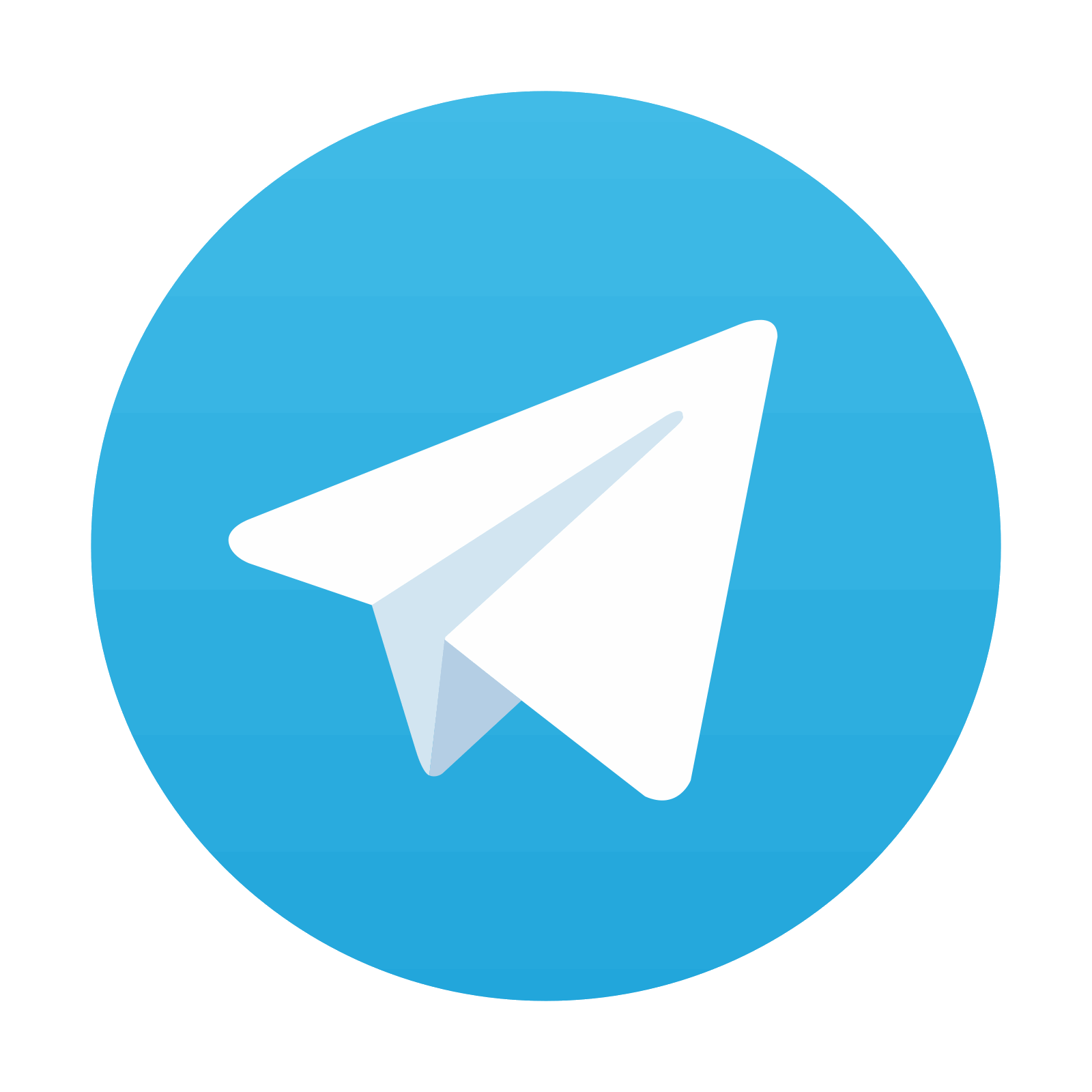
Stay updated, free articles. Join our Telegram channel
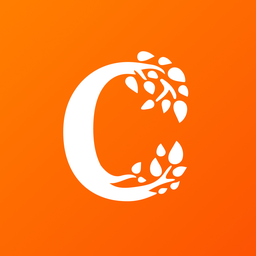
Full access? Get Clinical Tree
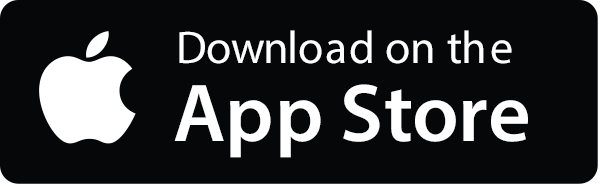
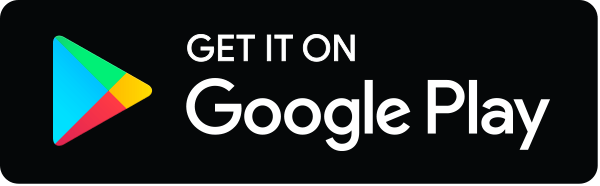