Fig. 5.1
IVUS-derived plaque evident in angiographically normal segments. A coronary angiogram showing minimal atherosclerotic disease within the mid portion of the left circumflex artery. Inset shows the corresponding IVUS cross-sectional view highlighting significant plaque burden (Adapted from Puri R, Tuzcua EM, Nissen SE, et al. Exploring coronary atherosclerosis with intravascular imaging. Int J Cardiol. 2013. doi.org/10.1016/j.ijcard.2013.03.024. With permission from Elsevier)
The high-resolution images attained from IVUS allow for the accurate identification of the lumen–plaque (or lumen–intima) interface, as well as the EEM. Allowing for the negligible thickness of the media, guidelines issued by the American College of Cardiology and European Society of Cardiology have endorsed the calculation of the area between the EEM and lumen edges as being the area occupied by plaque [22]. A constant automated transducer pull-back speed permits the volumetric quantification of atheroma burden with IVUS (Figs. 5.2 and 5.3). The ability to image anatomically matched arterial segments at different time points also provided the opportunity to accurately measure the effect of various therapeutic strategies on disease progression (Fig. 5.4).
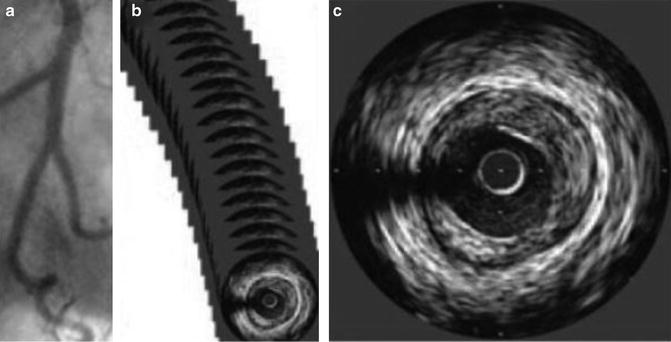
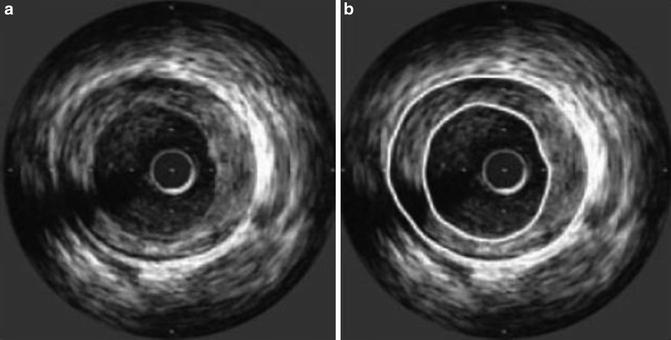
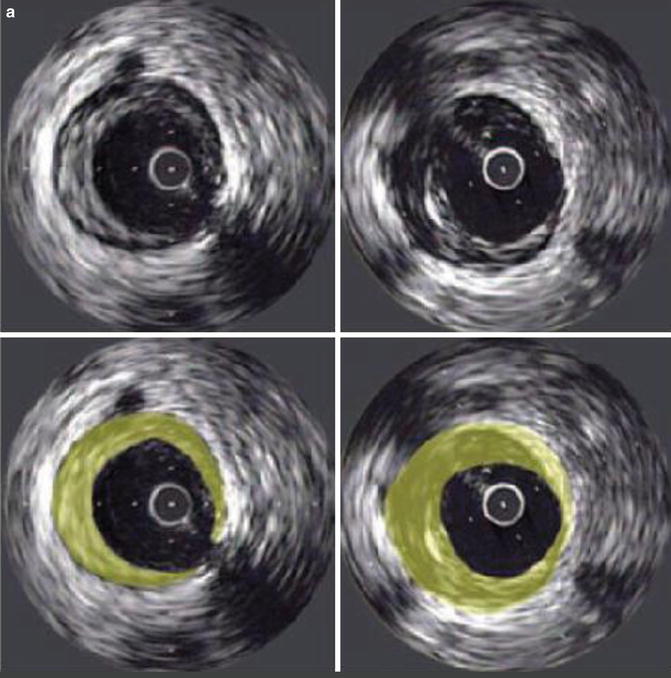
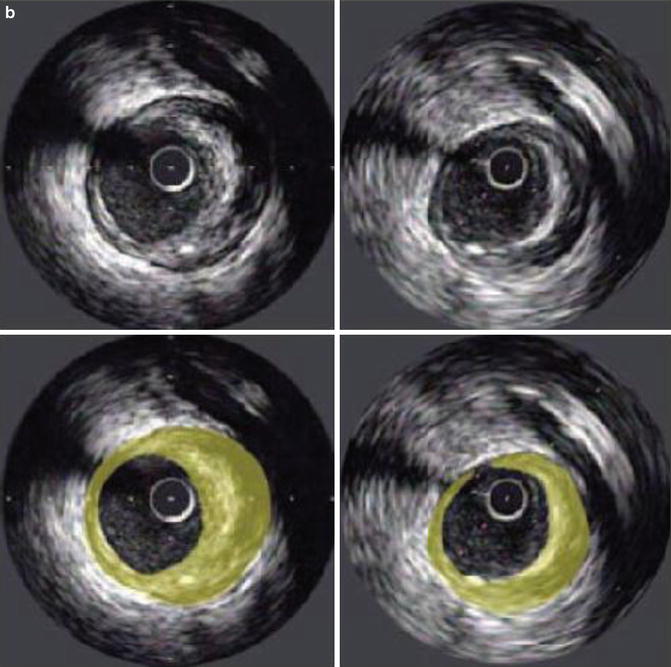
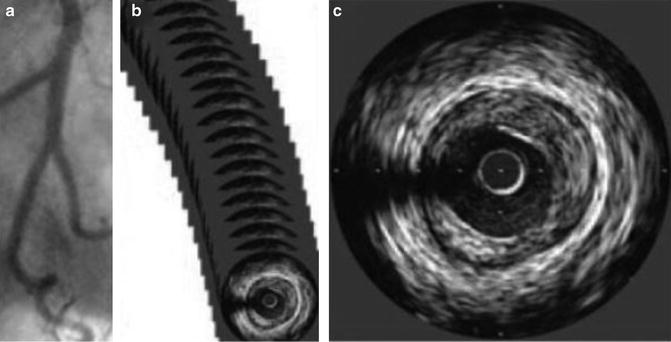
Fig. 5.2
Generation of images by intravascular ultrasound. Pullback of the ultrasound transducer through the artery (a) generates a series of tomographic images (b). Images separated by 1-mm intervals are then used for measurements (c) (Adapted from Nicholls SJ, Sipahi I, Schoenhagen P, et al. Application of intravascular ultrasound in anti-atherosclerotic drug development. Nat Rev Drug Discov. 2006;5(6):485–92. With permission from Nature Publishing Group)
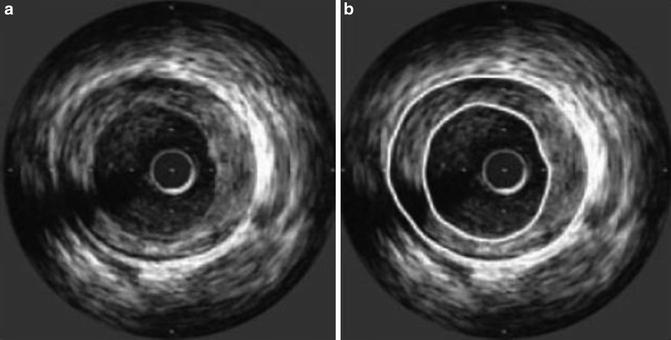
Fig. 5.3
Images from intravascular ultrasound. (a) Representative example of a cross-sectional tomographic image of a coronary artery acquired by intravascular ultrasound. (b) The panel illustrates the standard measurements that are made by manual planimetry of the leading edges of the external elastic membrane (outer circle) and lumen (inner circle). The area between these leading edges represents the plaque area (Adapted from Nicholls SJ, Sipahi I, Schoenhagen P, et al. Application of intravascular ultrasound in anti-atherosclerotic drug development. Nat Rev Drug Discov. 2006;5(6):485–92. With permission from Nature Publishing Group)
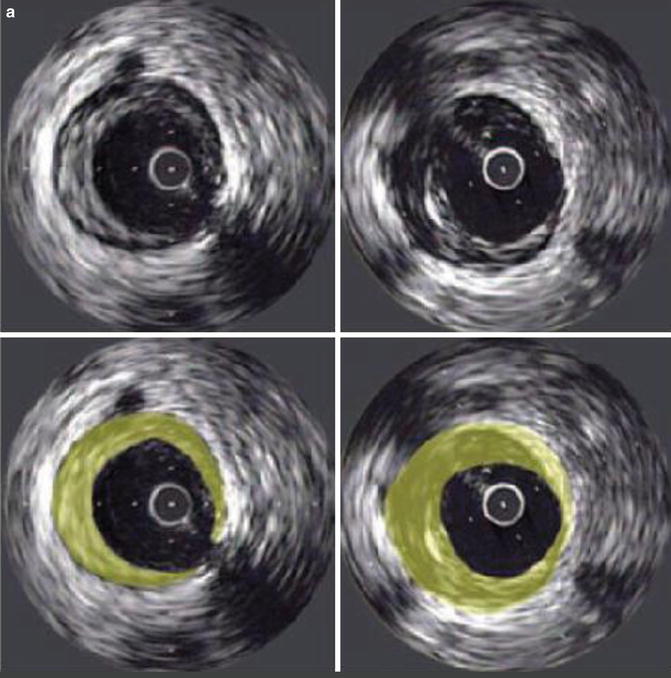
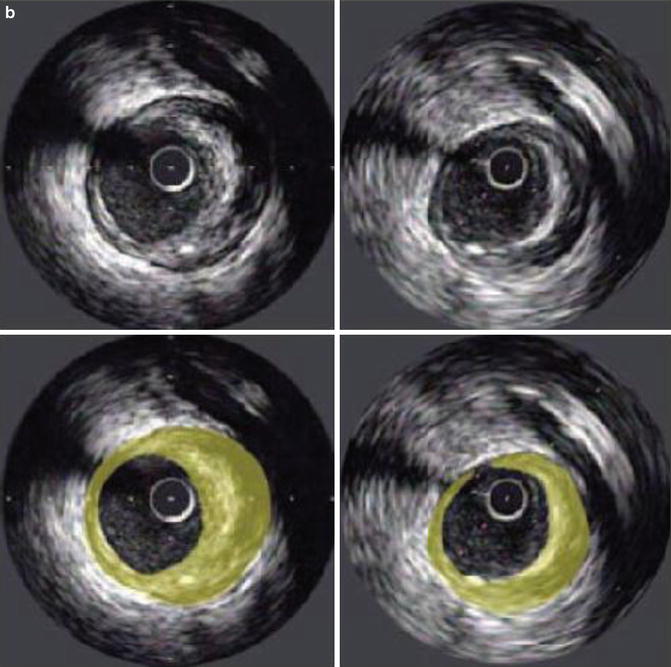
Fig. 5.4
Plaque progression and regression assessed by intravascular ultrasound. (a) Illustrative example of plaque progression at a matched site from IVUS studies performed in the same arterial segment a baseline (left panels) and follow-up (right panels).(b) Illustrative example of plaque regression at a matched site from IVUS studies performed in the same arterial segment at baseline (left panels) and follow-up (right panels). The shading in the lower panels highlights the plaque area at each time point (Adapted from Nicholls SJ, Sipahi I, Schoenhagen P, et al. Application of intravascular ultrasound in anti-atherosclerotic drug development. Nat Rev Drug Discov. 2006;5(6):485–92. With permission from Nature Publishing Group)
A number of experimental models and serial imaging studies in humans had previously suggested that atherosclerosis is a potentially reversible disease. In turn, numerous studies have been designed to assess the impact of favorably modifying one or more of the known traditional cardiovascular risk factors and the resulting influence upon the natural history of coronary atherosclerotic plaque. As a result, several drug classes have been tested in large-scale trials to determine whether they can halt the progression of atherosclerosis. A number of these trials have employed IVUS to detect changes in atheroma burden.
Low-Density Lipoprotein Cholesterol and Atherosclerosis Progression
Lowering serum low-density lipoprotein cholesterol (LDL-C) levels with statins has known anti-atherosclerotic effects, demonstrated in large-scale atherosclerosis imaging trials [5, 23–25], which support the consistent findings of reductions in hard clinical endpoints in both primary [26–28] and secondary disease prevention [29–33]. Most notably, these clinical benefits appear most pronounced in the setting of intensive LDL-C lowering [4]. However the precise mechanism(s) as to how statin therapy contributes to these benefits remains unclear. The degree of atheroma regression appears more modest than the magnitude of clinical benefit accrued from statins, as well as the residual burden of disease that persists during therapy. Prior angiographic studies had not shown consistent atherosclerosis regression with statin monotherapy to corroborate the profound impact that statin therapy had shown upon clinical event rates. With the known limitations of angiography in mind, trials were designed to test the hypothesis that intensive LDL-C lowering with statins would significantly alter the rate of coronary atheroma progression evaluated with serial IVUS.
A consistent observation in IVUS trials is the linear relationship between mean LDL-C levels achieved on statin therapy and the median progression–regression rate of atherosclerosis (Fig. 5.5). In the Reversing Atherosclerosis with Aggressive Lipid Lowering (REVERSAL) trial, 18 months of moderate LDL-C reduction (pravastatin 40 mg) resulting in a mean on-treatment LDL-C level of 110 mg/dL associated with significant disease progression [23]. Intensive LDL-C lowering (atorvastatin 80 mg) on the other hand, resulted in a mean on-treatment LDL-C level of 79 mg/dL, halting the natural progression of disease. Interestingly, despite there being a direct relationship between LDL-C lowering and slowing of disease progression, C-reactive protein lowering, a marker of systemic inflammation, also independently associated with less disease progression [34]. This suggests that non-cholesterol mediated, or pleiotropic effects of statins, are likely to be important in mediating the progression of disease.
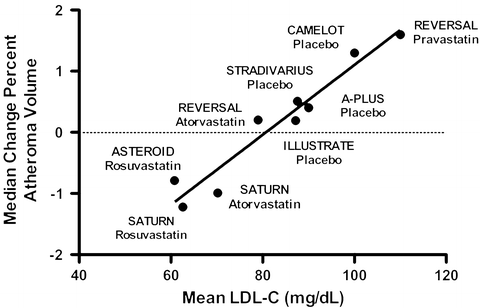
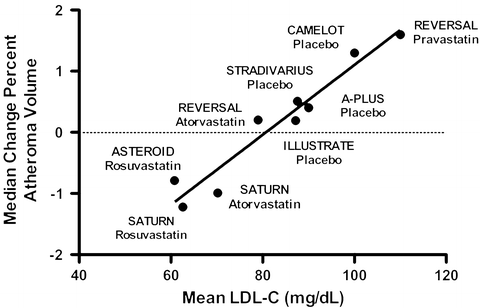
Fig. 5.5
The relationship between plaque regression and achieved LDL-C levels in clinical trials. Line of regression highlighting the relationship between on-treatment mean LDL-C vs. median change in atheroma volume (Adapted from Nicholls SJ, Ballantyne CM, Barter PJ, et al. Effect of Two Intensive Statin Regimens on Progression of Coronary Disease. N Eng J Med 2011;365:2078–87. With permission from Massachusetts Medical Society)
The ASTEROID (A study to evaluate the effect of rosuvastatin on IVUS-derived coronary atheroma burden) trial was designed to test the hypothesis that lowering LDL-C levels to below those achieved in REVERSAL might regress plaque. Unequivocal LDL-C reductions to a mean on-treatment level of 61 mg/dL were achieved with rosuvastatin 40 mg daily for 24 months. As predicted by the regression line, this degree of LDL-C lowering associated with significant plaque regression [24]. On the basis of these findings, SATURN (Study of Coronary Atheroma by Intravascular Ultrasound: Effect of Rosuvastatin Versus Atorvastatin) was performed to directly compare the anti-atherosclerotic efficacy of rosuvastatin 40 mg daily and atorvastatin 80 mg daily for 24 months [5]. Marked regression of coronary atherosclerosis was evident in each treatment group, following the achievement of very low on-treatment LDL-C levels (62 mg/dL vs. 70 mg/dL) in the rosuvastatin and atorvastatin arms, respectively, with two-thirds of the SATURN population demonstrating disease regression. These findings highlight the benefit of high-intensity statin therapy in patients with coronary artery disease, particularly when achieved LDL-C levels are consistent with or lower than those recommended by current treatment guidelines [35, 36]. It remains to be seen however, whether a ceiling effect of the magnitude of plaque regression exists, or whether further degrees of regression are achievable when on-treatment LDL-C levels are driven below those achieved in SATURN. Clinical trials are currently underway to test this hypothesis. The finding that a proportion of individuals continue to demonstrate disease progression despite significant LDL-C lowering with high-intensity statin therapy also highlights the multifactorial nature of the disease process [37], the need to globally intensify risk-factor control in these patients, as well as the importance of identifying novel anti-atherosclerotic strategies to tackle the residual risk and disease burden following statin therapy.
High-Density Lipoprotein Cholesterol and Atherosclerosis Progression
A number of animal and population-based studies have demonstrated the protective effects of high-density lipoprotein cholesterol (HDL-C) [38, 39]. The inverse relationship between HDL-C levels and cardiovascular risk holds true even in the setting of very low LDL-C [40]. A meta-analysis of several trials that employed serial IVUS measures of coronary atheroma volume identified that plaque regression was most likely to occur in patients who, despite achieve LDL-C levels below 87.5 mg/dL, also experienced an increase in HDL-C from baseline of at least 7.5 % [41]. As a result, considerable attention has focused upon developing biological strategies of elevating HDL-C levels and/or promoting HDL particle functionality.
IVUS-based trials, that have tested the anti-atherosclerotic efficacy of direct HDL infusions in humans, have collectively demonstrated safety and potential benefit. Rapid and large amounts of coronary atheroma regression was demonstrated in relatively few patients afflicted with acute coronary syndrome who underwent weekly intravenous infusions of reconstituted HDL, vs. placebo infusion, over a 5-week period [42]. Further analysis revealed that this degree of plaque regression occurred in concert with preservation of lumen size [43], indicative of reverse remodeling of the coronary arterial wall, most likely a result of rapid plaque delipidation. Similar, beneficial effects have been observed following infusion of apoA-1 particles or autologous, delipidated HDL particles [44, 45]. Whether these favorable effects upon the arterial wall will translate into clinical benefit, remains to be investigated.
The ability to substantially raise HDL-C levels stimulated enthusiasm for the development of cholesteryl ester transfer protein (CETP) inhibitors. This enzyme is responsible for the transfer of esterified cholesterol from HDL-C to atherogenic LDL-C particles in exchange for triglyceride [46]. This pathway seemed an attractive target, as inhibition of CETP would not only result in preventing the cholesterol enrichment of atherogenic lipoproteins, but also substantially raise HDL-C levels, with concomitant modest LDL-C lowering. To add support to this hypothesis, lower cardiovascular event rates have been observed in populations with a genetic predisposition for CETP inhibition, and elevated CETP levels associated with increasing risk of cardiovascular events [47, 48]. However, the first tested CETP inhibitor, torcetrapib, failed to alter the natural progression of coronary atherosclerosis [49], and a parallel run large-scale phase 4 clinical trial was prematurely terminated due to molecule-specific toxicity related to torcetrapib’s activation of the renin–angiotensin–aldosterone system [50]. A post hoc analysis however, revealed that those with the highest achieved HDL-C levels demonstrated atheroma regression, indicative of intact HDL functionality in mobilizing lipid from the coronary arterial wall in such patients [51]. Next-generation CETP inhibitors are subsequently under clinical investigation.
Another therapeutic approach involving HDL is to promote the generation of functional HDL particles in vivo, via the up-regulation of endogenous hepatic ApoA-1 synthesis. The theory being that this would result in the generation of nascent HDL particles that would have the ability to undertake the variety of known anti-atherosclerotic functions of HDL, such as reverse cholesterol transport and anti-inflammatory effects. The implications of an oral ApoA-1 inducer (RVX-208) upon the progression of coronary plaque is currently being tested in a serial IVUS study called ASSURE (ApoA-1 Synthesis Stimulation and Intravascular Ultrasound for Coronary Atheroma Regression Evaluation) [52].
Blood Pressure
Little is known about the direct anti-atherosclerotic effects of systemic blood pressure lowering, with a study employing serial carotid intima-medial thickening measurements demonstrating attenuation of disease progression following commencement of antihypertensive therapy [53]. Contrary to current national blood pressure lowering guidelines, it remains unclear as to what the optimal or target blood pressure should be in patients afflicted with coronary artery disease. The Comparison of Amlodipine vs. Enalapril to Limit Occurrences of Thrombosis (CAMELOT) trial was designed to assess the effect of blood pressure reduction with amlodipine or enalapril (compared to placebo) in patients with coronary artery disease [54]. An embedded IVUS sub-study within CAMELOT demonstrated that blood pressure lowering with amlodipine of the magnitude of 5/3 mmHg corroborated a halting of atheroma progression, compared with the placebo group whose atheroma burden progressed significantly. A further analyses revealed a direct relationship between the degree of systolic blood pressure lowering and plaque volume, with a trend towards plaque regression in patients with a systolic BP < 120 mmHg [55]. A post hoc analysis examined the effect of intensive control of both LDL and blood pressure upon atheroma progression in coronary artery disease patients. Patients with on-treatment serum LDL levels ≤70 mg/dL and systolic blood pressure ≤120 mmHg experienced less disease progression and more frequent atheroma regression [56]. These findings suggest the need for such patients to have their blood pressure lowered to levels below those endorsed by current national blood pressure guidelines. As such, there is currently no consensus of the optimal blood pressure target in patients with demonstrable coronary disease, particularly when guidelines currently recommend a treatment goal of systolic blood pressure of <140 mmHg.
Diabetes and Obesity
As the incidence of diabetes mellitus continues to rise, parallel increases in the rates of diabetic atherosclerotic vascular disease are projected to impart major health and socioeconomic challenges for authorities worldwide. Atherosclerosis is the predominant disease phenotype in diabetic individuals, particularly within the coronary, cerebrovascular, and peripheral arterial territories. Diabetic individuals display progressive coronary atherosclerosis, despite LDL-C lowering [57], emphasizing the importance of evaluating novel therapies that reduce the burden and progression of diabetic atherosclerosis. Whilst controversy exists regarding the safety of rosiglitazone [58], IVUS has yielded important mechanistic insights into the anti-atherogenic effects of the PPAR-γ agonist pioglitazone in diabetic patients with coronary artery disease. PERISCOPE (Pioglitazone Effect on Regression of Intravascular Sonographic Coronary Obstruction Prospective Evaluation) was a trial that compared the effects of pioglitazone to the insulin secretagogue, glimepiride, upon atherosclerosis progression. The pioglitazone group demonstrated a significantly lower rate of plaque progression when compared to the glimepiride group, who demonstrated plaque progression. These results were supported by the clinical results from the PROACTIVE (Prospective Pioglitazone Clinical Trial In Macrovascular Events), a trial that demonstrated a reduction in hard clinical endpoints with pioglitazone [59].
The increasing prevalence of abdominal obesity is a major factor for the worldwide increase in metabolic syndrome, insulin resistance, and atherosclerotic cardiovascular disease. The Strategy To Reduce Atherosclerosis Development Involving Administration of Rimonabont—the Intravascular Ultrasound Study (STRADIVARIUS) tested the hypothesis that a medication that selectively antagonized the cannabinoid type 1 receptor over an 18-month period might reduce the progression of coronary artery disease in abdominally obese individuals with metabolic syndrome [60]. Rimonabant, however, had no significant effect upon the primary endpoint of change in percent atheroma volume (PAV) from baseline, but had a significant effect on the secondary endpoint, reduction of total atheroma volume (TAV). More frequent psychiatric adverse effects were also reported in the rimonabont group, despite more favorable changes in HDL-C and triglyceride levels, weight loss, and waist circumference reduction compared to the placebo group. Nevertheless, the lack of an overt anti-atherosclerotic effect from rimonabont led to cessation of development of this compound.
Non-Lipid Plaque-Modifying Therapies
IVUS has also been instrumental in outlining the effects of non-lipid modifying compounds on plaque. Inhibition of acyl-coenzyme A:cholesterol acyltransferase (ACAT) promised to be an exciting therapeutic target. The lack of a direct effect on serum LDL-C or HDL-C concentrations made the dose-finding exercise of the potential anti-atherosclerotic effects of this class of compound difficult. Imaging endpoints were thus considered the most appropriate technique for demonstration of mechanistic efficacy. Despite demonstrative anti-atherosclerotic properties of ACAT inhibitors in animal models of disease, the failure to observe disease regression on serial IVUS with ACAT inhibition in two separate clinical trials resulted in halting the development programs of ACAT inhibitors [61, 62].
The mechanical strain of the arterial wall, coupled with local tissue deformation can be determined by the cross-correlation analysis of the radiofrequency IVUS signal, to derive localized strain maps of the arterial wall. The imputed degree of radial strain can be incorporated within the plaque area to determine the local elastography of the segment, or upon the luminal boundary (to a depth of 450 μm) to determine the local palpography of the respective plaque segment. As such, these elastic properties of the coronary arterial wall have been proposed to predict plaque vulnerability [63]. Subsequently, the Integrated Biomarker and Imaging (IBIS-2) trial was designed to assess for changes in plaque deformability (utilizing IVUS-palpography) in response to the lipoprotein-associated phospholipase A2 inhibitor, darapladib [64]. This was the first drug-intervention trial designed to explore if a therapeutic intervention could modulate plaque composition, and subsequent plaque phenotype. Although it was postulated that darapladib would lower the deformability/strain of coronary atherosclerotic plaques, the results of this trial failed to show any overall significant effect of darapladib upon plaque mechanical strain, despite changes in atheroma composition, which was an exploratory endpoint. The total atheroma burden in the treatment group also remained unchanged, questioning the anti-atherosclerotic potential of this compound, although further clinical trials are currently underway to assess the anti-atherosclerotic and clinical efficacy of this compound.
Cardiac Allograft Vasculopathy
IVUS has also yielded significant insights into the pathogenesis and modulation of cardiac allograft vasculopathy, the single greatest determinant of allograft failure and overall mortality within this patient group. Moreover, serial IVUS imaging has been employed to assess the progression of cardiac allograft vasculopathy [65]. Everolimus-based immunosuppressive therapy following cardiac transplantation was demonstrated to show a beneficial impact upon the rate of progression of intimal thickening within cardiac allograft recipients [66]. Moreover, this was associated with fewer episodes of rejection and the need for repeat transplantation when compared to a standard azathioprine-based immunosuppressive regimen. A separate study identified the rate of intimal thickening seen on IVUS at the 1-year mark following cardiac transplantation to predict 5-year mortality [67].
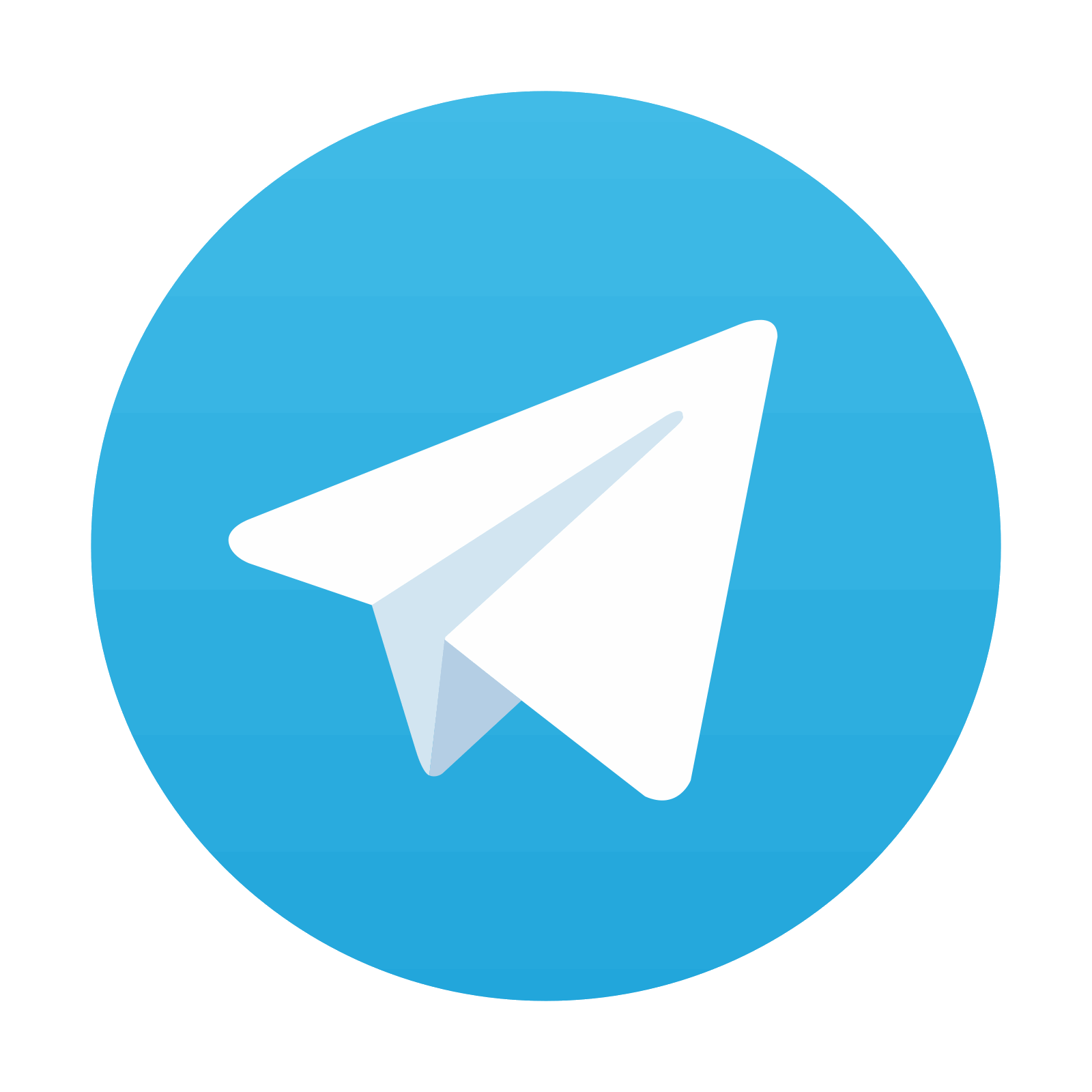
Stay updated, free articles. Join our Telegram channel
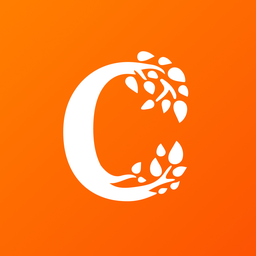
Full access? Get Clinical Tree
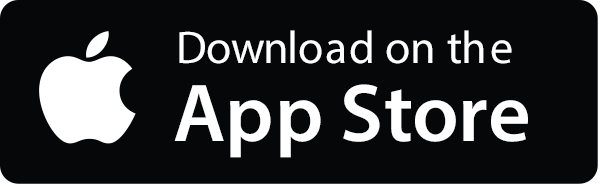
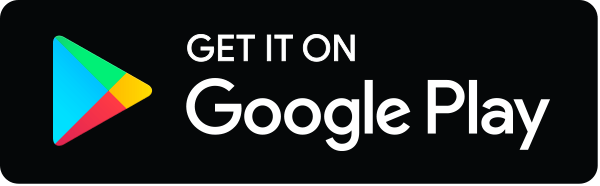