Fig. 14.1
A 61-year-old male with benign prostatic hypertrophy. Depiction of zonal prostate anatomy. (a–d) Axial T2-weighted images. (e) Parasagittal T2-weighted image and (f) coronal T2-weighted image. (a) At the level of seminal vesicles. (b) Base of prostate gland. (c) The midgland. (d) The apex. BL urinary bladder, CG central gland (central zone and transition zone), FS anterior fibromuscular stroma, PZ peripheral zone, SV seminal vesicle, U urethra, NV neurovascular bundle, V verumontanum

Fig. 14.2
(a) T2-weighted FSE image, (b) single axial H&E stained whole mount section and aligned 3 T multiparametric imaging data (c–i) from a 59-year-old patient with a PSA of 5.6 ng/ml and 1.5 cc 3+4 tumor in the right midgland. (a) T2-weighted MRI image showing a low signal intensity lesion (red arrows) in the right midgland. (c) Same T2-weighted image as in (a) showing the selected 0.16 cc 1H MRSI volume (bold white line) and a selected spectral array (white grid) and corresponding spectra very elevated choline and reduced citrate and polyamines within the right midgland (d and f) relative to the left midgland (d and e) in the region of cancer. (g) A calculated ADC map demonstrating a dramatic reduction in ADC in the region of cancer. (h) Dynamic contrast uptake and wash-out curve from a region of benign (green) and malignant prostate (green). (i) Calculated image of the slope of uptake of contrast agent demonstrating a much faster uptake in the region of cancer. It is very common that 1 or more biomarkers best identify cancer in one patient and a completely different set in another patient
Summary of Current Clinical Status
While T2 MRI remains a critical part of the prostate MR examination, particularly in regard to the assessment of the spread of cancer beyond the prostate, there remains a clear need for MR data that provide a functional assessment of the prostate in order to better discriminate prostate cancer from other benign pathologies and treatment effects, and to obtain an assessment of cancer aggressiveness within the prostate. The MR imaging detection and characterization of prostate cancer can be significantly improved through the addition of various functional sequences to the anatomic T2-weighted imaging such as MRSI, DWI, DCE imaging, quantitative T2 MRI, and by performing the multiparametric examination at higher magnetic field strengths (3 T) (Fig. 14.2). These techniques and the incremental clinical value they provide for the selection, guiding, and monitoring of focal therapy are discussed in the following sections.
Proton Magnetic Resonance Spectroscopic Imaging
Metabolic changes that are monitored by 1H Magnetic Resonance Spectroscopic Imaging (1H MRSI) have been shown to significantly improve the ability of MRI to detect and assess the location, volume, and aggressiveness of cancer within the prostate, as well as improve the assessment of extracapsular spread in patients [90]. It provides a noninvasive method of detecting small molecular biomarkers, specifically the metabolites choline, creatine, citrate, and polyamines, within the cytosol and extracellular spaces of the prostate and is always performed in conjunction with high-resolution anatomic imaging (Fig. 14.2).
Proton (1H) MRSI of the prostate is typically acquired using a combination of point resolved spectroscopy (PRESS) volume localization and three-dimensional (3D) chemical shift imaging (CSI) [91]. Robust acquisition of prostate 1H MRSI data has required the development of very accurate volume selection and efficient outer volume suppression techniques [92–94] (Fig. 14.2c). The resonances for citrate, choline, creatine, and polyamines occur at distinct frequencies or positions in the spectrum. The areas under these signals are related to the concentration of the respective metabolites, and changes in these concentrations can be used to identify cancer with high specificity [95]. In spectra taken from regions of prostate cancer (Fig. 14.2e), the citrate and polyamines are significantly reduced or absent, while choline is elevated relative to spectra taken from surrounding healthy peripheral zone tissue (Fig. 14.2d). The specificity of 1H MRSI for detecting, localizing, and characterizing the aggressiveness of prostate cancer relies on the unique metabolism of the prostate and the specific metabolic changes that occur with the evolution and progression of this disease, as described in the following sections.
Biochemical Rationale for Metabolic Changes Prior to Therapy
Healthy prostate epithelial cells have the specialized function of synthesizing and secreting large amounts of citrate that are dramatically reduced or lost in prostate cancer [96–99]. The loss of citrate in prostate cancer is intimately linked with changes in zinc levels that are extraordinarily high in healthy prostate epithelial cells [98, 100]. In healthy prostatic epithelial cells, the presence of high levels of zinc inhibits the enzyme aconitase, thereby preventing the oxidation of citrate in the Krebs’ cycle [98, 100]. Costello and Franklin have shown that these elevated levels of zinc are primarily due to increased expression of ZIP-type plasma membrane Zn uptake transporters (primarily Human ZIP-1) [99]. Human ZIP-1 is reduced and zinc levels are dramatically reduced in prostate cancer and the malignant epithelial cells, and there exists evidence that the loss of the capability to retain high levels of zinc is an important factor in the development and progression of prostate cancer [98, 100]. It is also believed that the transformation of prostate epithelial cells to citrate-oxidizing cells, which increases energy production capability, is essential to the process of malignancy and metastasis [97]. Additionally, both in vivo 1H MRSI studies [90, 101–104] and ex vivo HR-MAS spectroscopic studies of biopsy samples and surgically removed prostate tissues [105, 106] have shown that the degree of decrease in citrate correlates with Gleason grade.
The elevation of choline-containing metabolites phosphocholine (PC), glycerophosphocholine (GPC), and free choline (Cho)] and the over- and under-expression of key enzymes in the Kennedy cycle have been associated with the progression and therapeutic response of a variety of human cancers including prostate [90]. Specifically, HR-MAS spectroscopic studies of ex vivo surgical and biopsy prostate tissues demonstrated elevated levels of ethanolamine and choline containing compounds and that elevated PC and GPC were the most robust predictors of prostate cancer presence [107], and both in vivo 1H MRSI studies [90, 101–104] and ex vivo HR-MAS spectroscopic studies [105, 106] have shown that the degree of elevation of choline containing metabolites (PC and GPC) correlates with Gleason grade.
Healthy prostate epithelial cells also contain very high concentrations of polyamines, particularly spermine [90, 108, 109]. Polyamines are dramatically reduced in prostate cancer due to changes in the levels of expression of genes that regulate polyamine metabolism [90, 108]. The fact that choline and citrate change in opposite directions has led to the (choline + creatine)/citrate ratio (CC/C) being one of the most widely used metabolic biomarkers for detecting prostate cancer (54), and has been used in the majority of the current ≈ 320 publications on prostate MRI/MRSI [110]. It has also been demonstrated that when the CC/C ratio was ≥ 3 standard deviations above the normal value, there was minimal overlap between spectroscopic voxels from regions of cancer and healthy peripheral zone tissues [95], and the magnitude of elevation of the CC/C ratio has shown a correlation with cancer grade [90, 101, 111].
Biochemical Rationale for Metabolic Changes After Focal Therapy
To date, studies investigating 1H MRSI changes after therapy have focused on systemic therapy or the treatment of the entire prostate, rather than the ablation of dominant cancer foci within the prostate. These studies have indicated that the spectroscopic criteria used to identify residual/recurrent prostate cancer need to be adjusted due to a time-dependent loss of prostate metabolites following therapy. Specifically, time-dependent metabolic changes after androgen deprivation therapy (ADT), a common systemic therapy, have been studied using 1H MRSI [112–114]. ADT is the cornerstone of the systemic management of prostate cancer [115], and patients who succumb to prostate cancer typically do so only after ADT has ceased to be effective. ADT can take several forms—including medical castration with LHRH agonists, first-generation anti-androgens, 5-alpha-reductase inhibitors, or various combinations of these therapeutics—and can last for years. It affects both healthy and malignant prostate cells, inducing apoptosis and resulting in increasing amounts of tissue atrophy with duration of therapy [116]. Prostatic citrate production and secretion have been shown to be regulated by androgens [96], and an early dramatic reduction of citrate and polyamines after initiation of complete hormonal blockade has been observed by 1H MRSI [112–114]. There was slower loss of choline and creatine with increasing duration of hormone deprivation therapy [112]. This loss of prostatic metabolites correlates with the presence of tissue atrophy and has been considered a noninvasive indicator of effective therapy [113]. Time-dependent metabolic changes after ADT are of particular importance for selecting patients for focal therapy since many of these patients will be taking 5-alpha-reductase inhibitors, a form of ADT, which can result in significant changes in metabolism. In a recent serial multiparametric (T2-MRI, DWI, 1H MRSI) MR study of prostate cancer patients (N = 9) on active surveillance taking dutasteride (3.5 mg once-daily), 1H MRSI interpretation was confounded by the observation that citrate and polyamines decreased in healthy tissues while on dutasteride [117]. Thus, there was a bias to label healthy regions as likely cancer after dutasteride use. As the most dramatic changes in citrate and polyamines occurred in the first month, the one-month 1H MRSI measures provided an improved estimate of cancer presence and volume, particularly for small cancers mixed with healthy tissue, which was the case for this early stage patient cohort. Similar time-dependent reductions in prostate metabolites have also been observed after external bean radiation and brachytherapy of the entire prostate [118, 119].
Studies have also demonstrated the ability of MRI/1H MRSI to discriminate residual or recurrent prostate cancer from residual benign tissue and atrophic/necrotic tissue after cryosurgery [120–122], hormone deprivation therapy [112, 114], and radiation therapy [118, 123]. These studies have relied on elevated choline to creatine as a metabolic marker for prostate cancer since polyamines and citrate tend to disappear early after therapy in both residual healthy and malignant tissues (Fig. 14.3). Figure 14.3 shows a patient 9 years after external beam radiation therapy of the entire prostate. Consistent with effective radiation therapy, there were a number of spectroscopic voxels that demonstrated a complete loss of all prostate metabolites (metabolic atrophy). However, residual metabolic abnormalities (choline to creatine ≥ 1.5) persisted in the left midgland and this region was later confirmed as residual cancer by TRUS-guided biopsy. Two published MRI/1H MRSI studies have demonstrated that three or more consecutive voxels having choline/creatine >1.5 resulted in the ability to predict the presence of cancer after radiation therapy with an accuracy of ≈ 80% [124, 125]. In the case of focal therapy, regions of untreated benign tissue, surrounding the treated cancerous lesions, should demonstrate high levels of citrate and polyamines. In this scenario, the choline +creatine/citrate ratio would still be useful for the preservation of surrounding healthy prostate tissues surrounding the ablated region of cancer. The detection of residual cancer at an early stage following treatment and the ability to monitor the time course of therapeutic response would allow earlier intervention with additional salvage therapy [27, 126–131]. Alternatively, the lack of metabolic evidence of residual cancer after focal therapy could be used to demonstrate therapeutic success. This is a clinically important determination since residual benign tissue in the prostate will result in a nonzero serum PSA value, thereby diminishing its utility for predicting cancer presence relative to complete prostate therapeutic approaches such as radical prostatectomy.


Fig. 14.3
Example of a patient with a rising PSA of 2.04 at the time of the multiparametric MR examination which is up from a nadir of <0.01 after EBRT and neo-adjuvant hormone therapy. He obtained this multiparametric 3 T staging examination prior to a TRUS-guided biopsy. The multiparametric imaging examination demonstrated a large volume of aggressive appearing metabolic abnormality within the right midgland and apex with a corresponding region of suspicious T2 reduction (top left), dramatically reduced
(top right), elevated choline to creatine in 12 consecutive voxels (middle right) and more dramatic contrast agent uptake and washout in regions of residual tumor (yellow) compared to atrophic tissue (green) (bottom right). The TRUS-guided biopsy demonstrated recurrent cancer (RM—50% G4+4, and RA—67% G4+4) in the same locations as the imaging abnormality and the patient received high dose rate salvage brachytherapy

Summary of Current Clinical Status
1H MRSI provides unique metabolic information about the evolution and progression of prostate cancer and its response to therapy, and as described below, the addition of this metabolic information to high spatial resolution anatomic information provided by MRI shows great promise in aiding in the selection, targeting, and monitoring of prostate cancer focal therapy. However, the results of the ACRIN trial 6659 of 1.5 T MRI/1H MRSI of prostate cancer, as well as a number of publications, have demonstrated a clear clinical need to further improve the accuracy of the 1.5 T MRI/1H MRSI examination, particularly when trying to risk stratify an early stage prostate cancer patient population prior to focal therapy. The ACRIN multi-institutional study showed that MRI alone and combined 1H MRI/MRSI were of similar and modest accuracy (AUC of 0.60 for MRI and 0.58 for combined MRI/MRSI) in the localization of peripheral zone prostate cancer, and it did not confirm the primary hypothesis that the addition of 1H MRSI to MRI would improve tumor localization. This hypothesis was based on a large number of prior single institution studies demonstrating that the addition of 1H MRSI to MRI improves tumor localization [59, 132], tumor volume estimation [133], tumor staging for less experienced readers [87], and tissue characterization [90, 101, 111]. Given the preponderance of evidence that 1H MRSI adds value to MRI in the evaluation of prostate cancer, it is reasonable to ask why the ACRIN trial study did not demonstrate such a benefit? There are several possible reasons. The ACRIN study population consisted of a contemporary series of North American patients undergoing radical prostatectomy, involving predominantly low-risk patients with small volume, low-grade disease. Many of the tumors pathologically detected in the ACRIN study were at or below the current spatial resolution of 1.5 T 1H MRSI (≤ 0.5 cc) [101, 133]. Additionally, the application of 1H MRSI to organs outside of the brain is technically very challenging, and the ACRIN study involved dissemination of endorectal 1H MRSI to multiple sites and readers that had limited or no previous experience with it. That ACRIN successfully achieved this requirement for the study is a nontrivial accomplishment, and it is noteworthy that sites obtained studies of generally good spectral quality despite limited experience on a small number of test cases. Nevertheless, there were definite differences in the quality of 1H MRSI data provided by the participating institutions. Additionally, the clinical translation of 1.5 T 1H MRSI has been limited by insufficient spatial resolution to resolve small, low-grade cancers, relatively long acquisition times (17 min), and by technical issues. At 3 T, spatial resolution of 1H MRSI can be increased ≈ 2-fold (0.16 cc, ≈ 5 mm on a side) and the spectral data acquired in 8 min with better spectral signal to noise as compared to 0.34 cc 1H MRSI spectra acquired at 1.5 T in 17 min (Fig. 14.1c, d, e) [71]. Robust acquisition of 1H MRSI has required the development of very specialized data acquisition techniques and protocols [134]. Through the use of an inflatable endorectal coil with a compound that matches the susceptibility of tissue and a pulse sequence (MLEV-PRESS) specifically designed for prostate spectroscopy at 3 T [71], high spatial and temporal resolution 3D MRI/1H MRSI data can be obtained. Due to the pathologic and biologic complexity of prostate cancer, the addition of other high spatial resolution functional information to the MR staging examination will be key to patient selection and targeting focal therapy.
Diffusion-Weighted Imaging (DWI)
With the advent of hardware and software capable of acquiring single shot images, and ways to reduce the magnetic susceptability at the air tissue interface of the rectum, diffusion-weighted MR imaging has become not only feasible but more widely used and potentially a powerful clinical imaging technique for imaging prostate cancer [135]. Prostate DWI is most often performed using a single shot echo planar imaging sequence [40, 52, 136] but have also been performed using a single shot fast spin echo sequence [137] and line scan diffusion imaging techniques [63, 138]. DWI is sensitive to the motion of water molecules at microscopic spatial scales within biological tissues, and can provide unique information about microscopic tissue compartments, structural anisotropy, and the pathology of tissues [139–142]. The rate of diffusion in tissues is lower than in free solution and is described by an apparent diffusion coefficient (ADC), which largely depends on the number, permeabilities, and separation of barriers that a diffusing water molecule encounters, and variations in ADC have been shown to roughly correlate inversely with tissue cellularity [135, 143]. The MR ADC has been shown to be lower in prostate cancer than in surrounding benign prostate tissues (Fig. 14.1g). The loss of normal ductal architecture and increased cellularity that occurs with prostate cancer result in a smaller extracellular space and corresponding reduction in ADC [144]. However overlap exists between individual benign and malignant peripheral zone prostate tissues, with typical ADC values ranging from 2.0 to 1.4 and 1.6 to 0.9 × 10−3 mm2/s, respectively [135, 145]. ADC values in the central gland (central and transition zones) are reduced relatively to the peripheral zone, typcially ranging from 1.7 to 1.3 × 10−3 mm2/s, and central gland tumor ADCs on average are also slightly lower than those in the peripheral zone, 1.4 to 0.8 × 10−3 and 1.6 to 0.9 × 10−3 mm2/s, respectively [135, 145]. In particular, there is significant overlap between the low ADC values obseved for predominately stromal BPH and tumors within the central gland. However, DWI has still proven clinically useful in detecting central gland tumors, which are often missed on TRUS-guided biopsies (see Fig. 14.6a) [146]. MR diffusion has also recently shown promise for reflecting the pathologic grade of prostate cancer, with lower ADC values found in higher Gleason grade cancers [146–149]. For example, in one study ADC values decreased from 1.2 × 10−3 mm2/s in Gleason score 6 tumors to 0.8 × 10−3 mm2/s in Gleason score 8 prostate cancers [148], and in another study ADC decreased from 1.08 ± 0.02 × 10−3 mm2/s in cancers with Gleason score ≤ 6 to 0.94 ± 0.03 × 10−3 mm2/s for Gleason 4+3 cancer [149].
It has been shown that the addition of DWI to T2-weighted imaging increased the accuarcy of cancer diagnosis over T2-weighted imaging alone. Kim et al., investigating 3-T diffusion-weighted MR imaging in 36 patients, but without an endorectal coil, found that the combination of diffusion-weighted MR imaging and T2-weighted MR imaging led to an improvement in the area under the receiver operating characteristic (ROC) curve from 0.61 to 0.88 [150]. A pilot study of 50 patients with up to 2-year follow-up by Morgan et al. demonstrated that DW-MRI has potential for monitoring patients with early prostate cancer who opt for active surveillance [151]. Specifically, a 10% reduction in tumor ADC indicated progression with a 93% sensitivity and 40% specificity (A(z) of ROC curve = 0.68) [151]. The ability to detect, localize, and monitor early stage disease is key to focal therapy since this will likely be the target patient population for most focal therapuetic approaches.
It has also been shown that exposure of tumors to both chemotherapy and radiotherapy consistently leads to measurable increases in water diffusion in cases of favorable treatment response; however, regions of cancer still remained lower than surrounding benign and atrophic tissues [152, 153]. After radiation therapy, the mean ADC values of the biopsy-proven cancer areas (0.98 ± 0.23 × 10–3 mm2/s) were shown to be significantly lower than those of benign tissue (1.60 ± 0.21 × 10–3 mm2/s). A significantly greater area under the ROC curve (Az) was determined for combined T2 MRI and DWI (Az = 0.879, P < 0.01) compared to T2 MRI alone (Az = 0.612). Preliminary study also showed that DWI has potential for detecting residual cancer after systemic ADT [153], and focal focused ultrasound therapy [154].
Summary of Current Clinical Status
High spatial resolution DWI images can be easily and robustly acquired within a matter of minutes and therefore easily added to an MR staging examination, and the production of ADC maps quickly and easily performed [155]. The ease of acquisition and analysis combined with initial findings demonstrating clinical utility has led to great excitement over DWI’s role in prostate cancer focal therapy. However, in spite of significant differences between malignant and benign tissues in the peripheral zone before and after therapy, there is variability between patients as well as overlap of ADC values between malignant and benign prostatic tissues. This is not suprising since benign pathhologies such as prostatis and predominately stromal benign prostatic hyperplasia are often associated with pathologic changes that would lead to decreases in the extracellular space and corresponding reduction in ADC. The quantitative nature of DWI is also appealing; however, there remains a need for the test–retest validation of the quantitation of ADC values. There is also initial data suggesting that while ADC is increased in regions of prostate cancer after therapy, it remains lower than surrounding regions of treated benign and atrophic tissues [152, 153], but more studies after therapy are necessary to establish DWI utility for identifying residual disease and predict clinical outcomes.
Dynamic Contrast-Enhanced MRI
DCE MRI is currently performed by injecting a small molecular weight MR contrast agent (Gadolinium (Gd)-DTPA) into the patient, typically via the antecubital vein, and measuring the increase in signal intensity on fast T1-weighted images of the prostate [156]. The MR contrast agent, Gd-DTPA, does not penetrate prostate cells but can collect in the extracellular space [157]. The rate of enhancement reflects the volume and permeability of the blood vessels bringing the contrast agent to the tissue. Once the contrast agent is mixed in the vasculature, the peak enhancement in the tissue is due to the size of the extracellular space in which the Gd-DTPA can accumulate (both the interstitium and the vasculature) [158, 159]. An additional factor that needs to be considered in interpreting DCE MRI of prostate cancer is the inability of Gd-DTPA to enter the ductal morphology of healthy glandular tissues, and its subsequent ability to access residual ductal morphology in regions of prostate cancer [160].
Numerous qualitative and quantitative methods to summarize the large amounts of DCE MRI data have been suggested. Qualitatively, the characteristics of the dynamic uptake and washout curves can be used to generate images that can be overlaid over the corresponding high spatial resolution anatomic images (Fig. 14.2i). Quantitatively, the MR data are fit to mathematical (pharmacokinetic) models of the tissue behavior. To determine underlying physiologic parameters with these models, other tissue parameters must be measured or assumed such as the concentration of the contrast agent in the vasculature, referred to as the arterial input function (AIF), and the original T1 of the tissue. Modeling offers great benefits in terms of understanding physiology and in allowing comparisons across patients, scanners, and days. Pharmacokinetic modeling of DCE MRI data is based upon the work of Tofts and Kermode [157–159]. In this, the contrast agent is assumed to diffuse out of blood vessels into the extracellular, extravascular space (EES) based upon the concentration gradient and simple diffusion. Concentrations within compartments are assumed to be constant and parameters time invariant. Water throughout the voxel studied is presumed to interact with the Gd causing a decrease in T1 relaxation time, with there being a fast exchange of protons. The concentration of Gd in the tissue is modeled as in the extended Tofts–Kermode model [158]. SPGR signals are fit to the modeled equations and the parameters determined: (1) K trans (the transfer of the contrast agent from the vasculature into the EES), (2) k ep (the transfer back to the blood), (3) v p (the blood plasma volume fraction), and (4) v e (the EES volume fraction = K trans/k ep). The 1/T1 is assumed to linearly increase with the tissue concentration of gadolinium.
A number of groups have used DCE MRI to characterize differences between cancer and noncancerous prostate tissues [37, 66, 144, 156, 160–183]. These typically find cancer to have a faster uptake and higher enhancement than healthy tissues. For example, in one study, regions of cancer had a higher peak enhancement (P < 0.006), faster enhancement rate (P < 0.0008), and faster washout slope (P < 0.05) than normal PZ tissues. However, stromal BPH had the fastest enhancement rate (P < 0.003) of all prostate tissues and tended to have the greatest enhancement [176]. In another study, K trans and v e were positively correlated with cell density for all prostate tissues [175].
In comparisons of DCE MRI to histopathology post-prostatectomy, a rapidly increasing, then decreasing uptake curve or high v e, peak enhancement, slope or K trans has been used as criteria for cancer [37, 59, 162, 167]. Reported sensitivities for cancer detection were typically in the 50s and 60s percent while specificities were high, in the high 80s to low 90s in percent. In one study, dynamic contrast-enhanced MRI improved the performance of T2 MRI for cancer localization, and volume estimation was significantly improved by the addition of DCE MRI, with area under the ROC curve of 0.83 for the PZ and 0.81 for the TZ cancers (0.7 and 0.75, respectively, for the T2 MRI) [163]. The accuracy of detecting prostate cancer was higher for larger (foci > 0.5 mL) and higher grade prostate cancers (>10% of Gleason grade 4/5) [178]. DCE-MRI has also been found useful for the detection, localization, and volume assessment of anterior prostate cancers [174]. Moreover, in several preliminary DCE MRI studies, poorly differentiated tumors demonstrated the earliest and greatest rate of enhancement [165, 184, 185].
While there are very few studies of DCE MRI after prostate cancer therapy, there is a recent study demonstrating that peak enhancement was useful in detecting residual disease after external beam radiation therapy [164]. Specifically, on a sextant basis, DCE MRI had significantly better sensitivity (72% [21of 29] vs. 38% [11 of 29]), positive predictive value (46% [21 of 46] vs. 24% [11 of 45]) and negative predictive value (95% [144 of 152] vs. 88% [135 of 153] than T2 MRI. Specificities were high for both DCE MRI and T2w imaging (85% [144 of 169] vs. 80% [135 of 169]) [164]. There are also several preclinical studies indicating that anti-angiogenic therapies can be monitored by DCE MRI [186, 187], and one preliminary patient study. For prediction of local tumor progression of prostate cancer after high-intensity focused ultrasonic ablation, DCE-MRI was more sensitive than T2-weighted MRI with DWI, but T2-weighted MRI with DWI was more specific than DCE-MRI. The areas under the ROC curve for DCE-MRI and T2-weighted MRI with DWI were 0.77 and 0.77 for reader 1 and 0.85 and 0.81 for reader 2 [154].
Summary of Current Clinical Status
The superior sensitivity of DCE-MRI compared with T2 MRI, together with its high specificity, is arguably sufficient for its use for localizing cancerous lesions within the prostate for focal treatment [167]. Additionally, there are data suggesting that DCE-MRI can provide information on cancer aggressivenes, although there remains conflicting views as to how well DCE MRI parameters correlate with histological grade [188]. Similar to 1H MRSI, the acquistion and analysis of DCE-MRI data of the prostate are more challenging and takes longer than T2-weighted MRI and DWI. There also remains a need to determine whether qualitative vs. quantitative DCE-MRI parameters present the most robust predictive value and for the test–retest validation of DCE-MRI results. There is also initial evidence that DCE-MRI may be used to detect residual cancer after therapy based on a more dramatic contrast uptake and washout compared to surrounding regions of atrophy and residual benign tissues, but this needs to be proven in a larger number of studies.
Quantitative T2-Weighted Imaging
Historically, the detection of prostate cancer has been based on the qualitative assessment of low signal intensity on T2-weighted images by experienced radiologists. However, recently the ability of quantitative T2-mapping of the prostate to provide an improved cancer detection and volume estimation has been investigated. Generation of T2 maps require the collection of a series of T2-weighted images with various echo times. Signal decay in each voxel can be fitted by using a weighted least squares approach to obtain the T2 relaxation time. A magnetization prepared sequence utilizing nonselective composite 180º pulses and MLEV phase cycling followed by multi-slice spiral imaging sequence has been developed to provide robust T2 relaxation measurement in vivo in a clinically reasonable amount of time [189]. Quantitative T2 studies have demonstrated significantly lower T2 values in tumor compared to normal prostate peripheral zone [63, 190] (Fig. 14.4). Furthermore, it was suggested that water T2 positively correlated with citrate concentration in the prostate [191]. The higher spatial resolution of T2-mapping compared to 1H MRSI could offer more accurate tumor detection and localization.


Fig. 14.4
Sixty-one-year-old prostate cancer patient with biopsy-proven cancer (G3+3, left apex, red rectangle). High-resolution T2-weighted FSE images (upper left) and MRSI (lower left) showed decrease signal intensity and elevated choline and reduced citrate in region of prostate cancer.
maps (upper right) and T2 maps (lower right) demonstrated a significantly decreased
(cancer: 0.154 mm2/s vs. Healthy: 2.17 mm2/s) and T2 relaxation time (Cancer: 94.5 ms vs. Healthy: 177 ms) in the region of cancer compared to benign tissues


Summary of Current Clinical Status
Quantitative T2 MRI studies can be easily added to a multiparametric examination, extending the examination by a matter of minutes, and T2 maps of the prostate generated. The quantitative nature of these studies could overcome some of the specificity limitations of T2-weighted MRI. However, the loss of ductal morphology in common benign pathologies (e.g., inflammation, stromal BPH) that coexist with prostate cancer will also reduce the specificity of quantitative T2 MRI. Comparatively, much less is known about the clinical value of quantitative T2 MRI in the assessment of prostate cancer, and like the other MRI approaches discussed, there remains a need for the test–retest validation of quantitative T2 values obtained from the prostate.
Multiparametric MR—Selection of Focal Therapy Patients
To date, there are few studies that have attempted to combine all MR biomarkers from a multiparametric MR staging examination at diagnosis to achieve the best selection of focal therapy patients or to best target intra-prostatic cancer for treatment. However, there have been a number of clinical studies that have combined multiple MR parameters for the improved staging, and intra-glandular localization of prostate cancer. The published clinical applications of multiparametric MR imaging that could provide a significant impact on focal therapy patient selection and tumor targeting for focal therapy are reviewed in the following sections.
Predicting Organ-Confined Prostate Cancer
A more accurate prediction of organ-confined prostate cancer at the time of diagnosis would allow the determination of whether “active surveillance” or “focal therapy” is appropriate for a given patient. At 1.5 T MRI, it has been demonstrated that anatomical features on MRI such as bulging of the prostate, obliteration of the rectoprostatic angle, and asymmetry of the neurovascular bundle can predict extracapsular extension (ECE), with specificity (up to 95%) but with low sensitivity (38%) [192]. Two studies have suggested that the addition of 1H MRSI to MRI data can improve prostate cancer staging. In one study, it was found that tumor volume per lobe, estimated by 1H MRSI, was significantly (P < 0.01) higher in patients with ECE than in patients without ECE. Moreover the addition of an 1H MRSI estimate of tumor volume to high specificity MRI findings for ECE [192] improved the diagnostic accuracy and decreased the interobserver variability of MRI in the diagnosis of ECE of prostate cancer [87].
An important advance in the staging of prostate cancer has been the development of multivariable risk prediction instruments such as the Partin tables [193] the CAPRA score [194] and various nomograms, which combine clinical stage, serum PSA levels, and grade of biopsies results to predict at the time of diagnosis the pathologic stage of the cancer and insignificant disease, respectively [195, 196]. Two recent studies demonstrated that addition of MRI/1H MRSI findings could significantly improve the predictive ability of biopsy-based staging nomograms for predicting seminal vesicle invasion and organ-confined cancer. It was found that the nomogram plus endorectal MR imaging (0.87) had a significantly larger (P < 0.05) area under the curve than either endorectal MR imaging alone (0.76) or the nomogram alone (0.80) [47]. In another study of prostate cancer patients prior to radical prostatectomy, 1.5 T MRI/1H MRSI data were added to a nomogram for predicting (no ECE or SVI) in order to assess its incremental value. The contribution of MR/1H MRSI findings to predicting organ-confined prostate cancer was also significant [50].
Due to increased screening using serum prostate-specific antigen (PSA) and extended-template transrectal ultrasound (TRUS)-guided biopsies, thousands of patients with prostate cancer are being identified at an earlier and potentially more treatable stage [197]. However, the risk of overdetection, detecting a cancer that would not become clinically significant during that patient’s lifetime if left untreated, has been estimated to vary between 15% and 84% [198–200]. Therefore there is an increased interest in “active surveillance,” but clinical parameters alone are not sufficient to predict a benign disease course. The addition of MRI/1H MRSI data to clinical parameters has been shown to improve this prediction. In a study of 220 patients prior to surgery, the addition of MRI (AUC—0.803) and MRI/MRSI (AUC 0.854) to biopsy-based nomograms was found to significantly improve the prediction of indolent prostate cancer using a surgical definition of indolent disease (no ECE or SVI and <0.5 cm3 of cancer with no pattern 4 or 5 cancer) as the standard of reference [43]. In another study of 114 active surveillance patients who received a MRI/1H MRSI at baseline, with a median follow-up time of 59 months, it was demonstrated that patients with a lesion suspicious for cancer noted on a MRI/1H MRSI staging examination had a greater risk of Gleason upgrade at subsequent biopsy (HR 4.0; 95%CI 1.1–14.9) than patients without such a lesion [201] (Fig. 14.5). This study suggests that an abnormal MRI/1H MRSI prostate examination at diagnosis that is suspicious for cancer may confer an increased risk of Gleason upgrade at subsequent biopsy, and may help counseling potential candidates about active surveillance, and whether they are more appropriate candidates for focal therapy. Specifically, MR imaging could play a role in selecting the subset of patients with indolent or subclinical disease that would be more appropriately managed with active surveillance vs. patients with more aggressive localized disease that would benefit from focal therapy. Clearly the higher spatial resolution functional MR data provided by DWI and DCE MRI could further improve this assessment. More recently, the D’Amico risk stratification was found to correlate with the degree of suspicion of prostate cancer on multiparametric MRI [202].


Fig. 14.5
(a) A reception-profile corrected T2-weighted axial image (left) taken from a volume MRI/MRSI data set of a patient with PSA of 5.93 and a small volume of biopsy-proven cancer (2 mm of G3+3 in right midgland toward the apex). On T2 MRI there were no clear regions of hypointensity indicative of cancer in the right midgland and apex. The selected volume for spectroscopy (bold white box) and a portion of the 16 × 8 × 8 spectral phase encode grid was overlaid (fine white line) on the T2-weighted image (middle) with the corresponding 0.3 cm3 proton spectral array (right). 1H MRSI also demonstrated no evidence of cancer. Over 48 months after the baseline MRI/MRSI scan, the patient did not have a significant rise in PSA, or a change in biopsy results. (b) A reception-profile corrected T2-weighted axial image (left) taken from a volume MRI/MRSI data set of a patient with similar clinical parameters (PSA of 5.60 and 12 mm of G3+3 in left base). On T2 MRI, there was region of hypointensity (red arrows) in the left base, with a corresponding region of abnormal metabolism (elevated choline, reduce citrate and polyamines). On follow-up biopsy, there was an increase in the volume and grade of disease (28 mm of G3+4 in left base)
Multiparametric MR—Targeting Clinically Significant Cancer Within the Prostate
Ideally, MR targets need to be identified with high sensitivity and specificity [203], but at a minimum it is critical to maintain high specificity in this setting—focally treating a false positive tumor with an investigational device rather than conventional treatment is ethically problematic and clinically risky since the true site of dominant disease would presumably be untreated.
Localization of Intra-glandular Prostate Cancer
The reliable detection and localization of often small regions of clinically significant prostate cancer are therefore of critical therapeutic importance to focal ablative therapy since the patient population it will impact the most will be an early stage population [204]. T2-weighted MRI alone has demonstrated good sensitivity but poor specificity in detecting cancer in the prostate [62, 78, 205]. Recent estimates using T2-weighted sequences and endorectal coils vary from 60% to 96% [60]. The poor specificity is due to other benign pathologies (inflammation, stromal BPH) and therapy also causing a loss of ductal morphology and low T2 on MRI [206]. Additionally, infiltrating prostate cancer may not cause a reduction in normal glandular morphology and therefore will not be hypointense on MRI [206]. Similar to imaging at 1.5 T, T2-weighted image quality, prostate cancer localization and staging are significantly improved at 3 T with the use of an endorectal coil compared with a external phased array coil [207]. Identifying prostate cancer within the central gland is particularly difficult for MRI due to the overlap of T2-weighted signal intensity in predominately stromal BPH. In a recent study of 148 prostate cancer patients prior to radical prostatectomy, MR imaging alone detected zone prostate cancers with modest accuracy with areas under the reader operator curve (AUC) ranging from 0.73 to 0.75 [208].
The higher specificity of 1H MRSI to metabolically identify cancer can be used to improve the ability of MRI to identify the location and volume of cancer within the prostate [132, 133, 209–212]. A study of 53 biopsy-proven prostate cancer patients prior to radical prostatectomy and step-section pathologic examination demonstrated a significant improvement in cancer localization to a prostatic sextant (left and right—base, midgland, and apex) using combined MRI/1H MRSI vs. MRI alone [132]. A combined positive result from both MRI and MRSI indicated the presence of tumor with high specificity (91%), while high sensitivity (95%) was attained when either test alone indicated the presence of cancer [132]. The addition of positive sextant biopsy findings to concordant MRI/MRSI findings further increased the specificity (98%) of cancer localization [212]. However, more recent studies in early stage prostate cancer patients have indicated that combined 1.5 MRI/MRSI does poorly at detecting and localizing small low-grade tumors [101, 133, 209]. One study demonstrated that overall sensitivity of MR spectroscopic imaging was 56% for tumor detection, increasing from 44% in lesions with Gleason score of 3+3 to 89% in lesions with Gleason score greater than or equal to 4+4 [101]. The inability to detect small low-grade tumors by 1.5 T 1H MRSI is primarily due to the partial volume averaging of surrounding benign tissue in spectroscopic volumes containing cancer due to the relatively coarse spatial resolution of 1.5 T MRSI (0.34 cc, ≈ 7 mm on a side).
In a recent meta-analysis of the 1.5 T MRI/1H MRSI literature, 31 studies (1,765 patients) were identified in the literature; 16 studies (17 populations) with a total of 581 patients were suitable for meta-analysis [110]. Nine combined MRI/1H MRSI studies (10 populations) examining men with pathologically confirmed prostate cancer (297 patients; 1,518 specimens) had a pooled sensitivity and specificity on prostate subpart level of 68% (95% CI, 56–78%) and 85% (95% CI, 78–90%), respectively. Compared with patients at high risk for clinically relevant cancer (six studies), sensitivity was lower in low-risk patients (four studies) (58% [46–69%] vs. 74% [58–85%]; P > 0.05) but higher for specificity (91% [86–94%] vs. 78% [70–84%]; P < 0.01). Seven studies examining patients with suspected prostate cancer at combined MRI/MRSI (284 patients) had an overall pooled sensitivity and specificity of 82% (59–94%) and 88% (80–95%). In the low-risk group (five studies), these values were 75% (39–93%) and 91% (77–97%), respectively.
DWI and DCE images can be acquired within a matter of minutes at very high spatial resolution (0.9 × 1.8 × 4 mm3), allowing their addition to a clinically reasonable MRI/1H MRSI examination and potentially improving MR detection of small low-grade tumors [155]. DCE imaging at 1.5 T and 3.0 T demonstrated similar sensitivities (73% and 73%, respectively) and specificities (81% and 77%, respectively) for identifying cancer within the prostate [62, 213]. In another study of prostate cancer patients who received an MRI/1H MRSI/DCE examination prior to radical prostatectomy, it was demonstrated that reader accuracy in tumor detection was significantly (P < .01) better for 3D MRSI (AUC = 0.80) and DCE (AUC = 0.91) than T2-weighted imaging (AUC 0.68) [59]. However, no attempt was made to determine the accuracy when all 3 techniques were combined. In a recent study, a combination of T2-weighted imaging, DWI, DCE, and MRSI was used to identify the regions suspicious for prostate cancer that were biopsied under MR guidance. They showed that all areas that were determined to be suspicious or indeterminate on T2-weighted MRI were suspicious on at least one advanced imaging technique and that the combination of the 3 advanced techniques depicted more prostate cancer areas than the combination of any 2 of them [214]. 3 T DWI studies also demonstrated good sensitivity (84%) and specificity (80%) for identifying cancer within the prostate [56], and the overall accuracy (AUC = 0.89) was found to be better than that of T2 imaging (AUC = 0.82) [51]. A positive correlation was also found between 1H MRSI and DWI findings for prostate cancer [215]. Another study that utilized combined DWI and MRSI of 61 prostate cancer patients demonstrated that the combination (AUC = 0.85) performed significantly better than MRSI alone (AUC = 0.74; P = .005) and was also better than
alone (AUC = 0.81, P = .09) for differentiating between benign and malignant ROIs in the PZ [216].

Estimation of Intra-glandular Prostate Cancer Volume
The reliable assessment of the extent or volume of prostate cancer is also critical for MR-guided focal ablative therapy, and the ability to accomplish is challenging due to the relatively early stage population that will most likely be treated. Two recent studies suggest that MRI/1H MRSI and DCE imaging may noninvasively provide estimates of cancer volume at diagnosis. One study demonstrated that for nodules greater than 0.5 cm3, tumor volume measurements by MRI, 1H MRSI, and combined MRI and MRSI were all positively correlated with histopathologic volume (Pearson’s correlation coefficients of 0.49, 0.59, and 0.55, respectively), but only measurements by 1H MRSI and combined MRI/1H MRSI reached statistical significance (P < 0.05) [133]. The addition of 1H MRSI to MRI also increased the overall accuracy of prostate cancer tumor volume measurement, although measurement variability still limited consistent quantitative tumor volume estimation, particularly for small tumors (<0.5 cm3) [133]. Another study demonstrated that DCE imaging determined the volume of smaller foci of prostate cancer with greater overall accuracy than MRI/1H MRSI. Sensitivity, specificity, and positive and negative predictive values for cancer detection by DCE imaging were 77%, 91%, 86%, and 85% for foci >0.2 cc, and 90%, 88%, 77%, and 95% for foci greater than 0.5 cc, respectively [57]. In another study, the sensitivity and specificity of detecting intraprostatic prostate cancer by DCE MRI was determined to be 86% and 94%, respectively, with a ROC of 0.874 [178]. Mean volume of DCE-MRI detected and missed cancers were 2.44 mL (0.02–14.5) and 0.16 mL (0.005–2.4), respectively [178]. In a study of 42 patients, the addition of DWI to T2 MRI significantly improved the accuracy of prostate peripheral zone tumor volume measurement [217]. Specifically, using a ADC cutoff of 0.0016 mm2/s for cancer, the concordance correlation coefficient of combined T2-MRI and DWI MR imaging was significantly higher (P = .006) than that of T2-MRI alone.
Multiparametric MR-Guided Interventions
Multiparametric MR may identify and provide a more accurate localization of clinically significant cancer within the prostate, and can subsequently be used to directly target biopsies and therapy (Fig. 14.3). The development of the field of direct prostate MR-guided interventions (biopsy and therapy) began with the introduction of a low field (0.5 T) open MRI device at the Brigham & Women’s Hospital (Boston, MA) [218], in 1993. They utilized this low field open magnet and real-time image guidance to perform transperineal biopsies of 50 patients, with a 30% cancer positive sampling rate [219–222]. The joint team from Johns Hopkins University and the National Institutes of Health moved the MR-guided biopsy procedure to a closed-bore 1.5 T MRI scanner in order to acquire superior quality MR data for biopsy targeting. They initially used a transperineal biopsy approach similar to the open MRI low field approach, but which required moving the patient in and out of magnet. They used this approach to biopsy and place HDR brachytherapy catheters in four patients with intermediate to high-risk prostate cancer [223]. The same group also developed a transrectal biopsy approach using a remotely operated needle guide, in combination with an endorectal coil for image reception, and an associated biopsy planning and imaging control method [224]. They utilized this approach for MR-guided intraprostatic placement of gold fiducial markers (four procedures) and/or prostate biopsy (three procedures) to patients scheduled to receive a standard course of conformal external beam radiation therapy [225].
In the setting of focal therapy, a MRI targeted biopsy could be used prior to treatment to confirm the region for treatment, as well as the effectiveness of the focal therapy. Moreover, to successfully perform focal therapy, there must be real-time imaging guidance of the treatment. The approaches that have been clinically used for multiparametric MR-guided prostate biopsy and therapy are described in more detail below.
MR-Directed TRUS Biopsy
Numerous studies reported in the literature have used the results from multiparametric MR staging examinations, performed prior to the TRUS biopsy, to identify regions of MRI suspicious cancer for subsequent real-time TRUS-guided biopsy [3, 226–229]. This MRI targeted biopsy approach has been utilized in several studies of patients with prior negative biopsies, and rising PSA. These patients typically have cancers in locations such as the anterior aspect of the prostate that are difficult to biopsy or in patients with large prostates where the sampling error of TRUS-guided systematic biopsy approaches are reduced. Specifically, in two studies, the accuracy of cancer detection of MRI/1H MRSI targeted biopsy in men with a prior negative biopsy was reported to be ≈ 80% [226, 227]. Combining DCE-MRI with 1H MRSI demonstrated a further improvement to guide biopsy needles to cancer foci in patients with previously negative TRUS biopsy [230, 231]. The combination of 1H MRSI and DCE-MRI yielded 93.7% sensitivity, 90.7% specificity, 88.2% PPV, 95.1% NPV, and 90.9% accuracy in detecting prostate carcinoma in a prior negative biopsy population [231]. In addition to improving cancer detection, MRI targeted, TRUS-guided biopsies also improved estimates of cancer volume and pathologic grade compared to a TRUS-guided systematic biopsy approach [232]. Additionally, combined T2-weighted and DCE-MRI was shown to be useful for guiding TRUS biopsies toward areas containing recurrent cancer after HIFU [233] and radiation therapy [164]. It is clear that the MR-directed TRUS biopsy approach is limited by inaccuracy in targeting small tumors as there is guesswork involved in triangulating lesions seen on multiparametric MRI to ultrasound. To date studies have added MR targeted biopsies to a systematic TRUS biopsy protocol, and whether a targeted approach alone is clinically sufficient requires further study [229]. The guesswork involved with transferring the multiparametric MR identified foci of cancer onto ultrasound data can be overcome by performing MRI-guided biopsy within the bore of the magnet.
Direct MRI-Guided Biopsy
The recent development and implementation of MR-guided biopsy devices allow for a direct means of taking a biopsy of prostate cancer identified using multiparametric data. As shown in Fig. 14.6, the current commercially available MR-guided biopsy device (DynaTRIM, Invivo Corporation, Orlando, FL) uses a transrectal biopsy approach. The patient is placed in the prone position inside the magnet. A body phased array coil is placed on the patient’s lower back and abdomen, and a MR visible needle guide that is attached to the MR biopsy device is inserted into the rectum (Fig. 14.6). Based on imaging findings, the MR-compatible biopsy device is adjusted using software that interfaces with the scanner and manual adjusts in all three spatial dimensions to obtain a biopsy core from the region of interest within the prostate. The needle guide is tracked by fast anatomic MR imaging during the adjustment of the biopsy device. Several studies have demonstrated the clinical potential of MRI-guided biopsies for detecting clinically significant prostate cancer prior to therapy [234–239] and residual disease after therapy [182]. Prior to therapy, the detection rate of multiparametric 3 T magnetic resonance imaging-guided biopsy was 59% (40 of 68 cases), which was significantly greater than transrectal ultrasound (15%) and 37 of the 40 patients (93%) with positive biopsies were considered highly likely to harbor clinically significant disease [238]. In a study of 24 patients with rising PSA after external beam radiation therapy, multiparametric MR identified areas of residual/recurrent prostate cancer with a positive predictive value of 75% based on MR targeted biopsies of the MR suspicious area [182].


Fig. 14.6
This is a patient with a PSA of 6.02 and a prior negative biopsy. (a) T2-weighted FSE image depicts low signal mass (red arrows) with high choline to citrate ratio and reduced ADC at the anterior aspect of the left central gland, midgland level. Given relative inaccessability of this lesion on TRUS and history of negative biopsy, this patient obtained a MR-guided biopsy. (b) At the time of prostate biopsy, initial determination of the position of the probe is obtained by localizer and T2-weighted FSE image. This measures the coordinates of the biopsy guide (green) relative to the target determined on the staging examination (red arrows). Then the biopsy needle is projected onto the T2-weighted image and the coordinates of the target dialed on the biopsy guide and prostate re-imaged to locate the biopsy needle. Biopsy yielded a G(3+3) tumor in the anterior left central gland
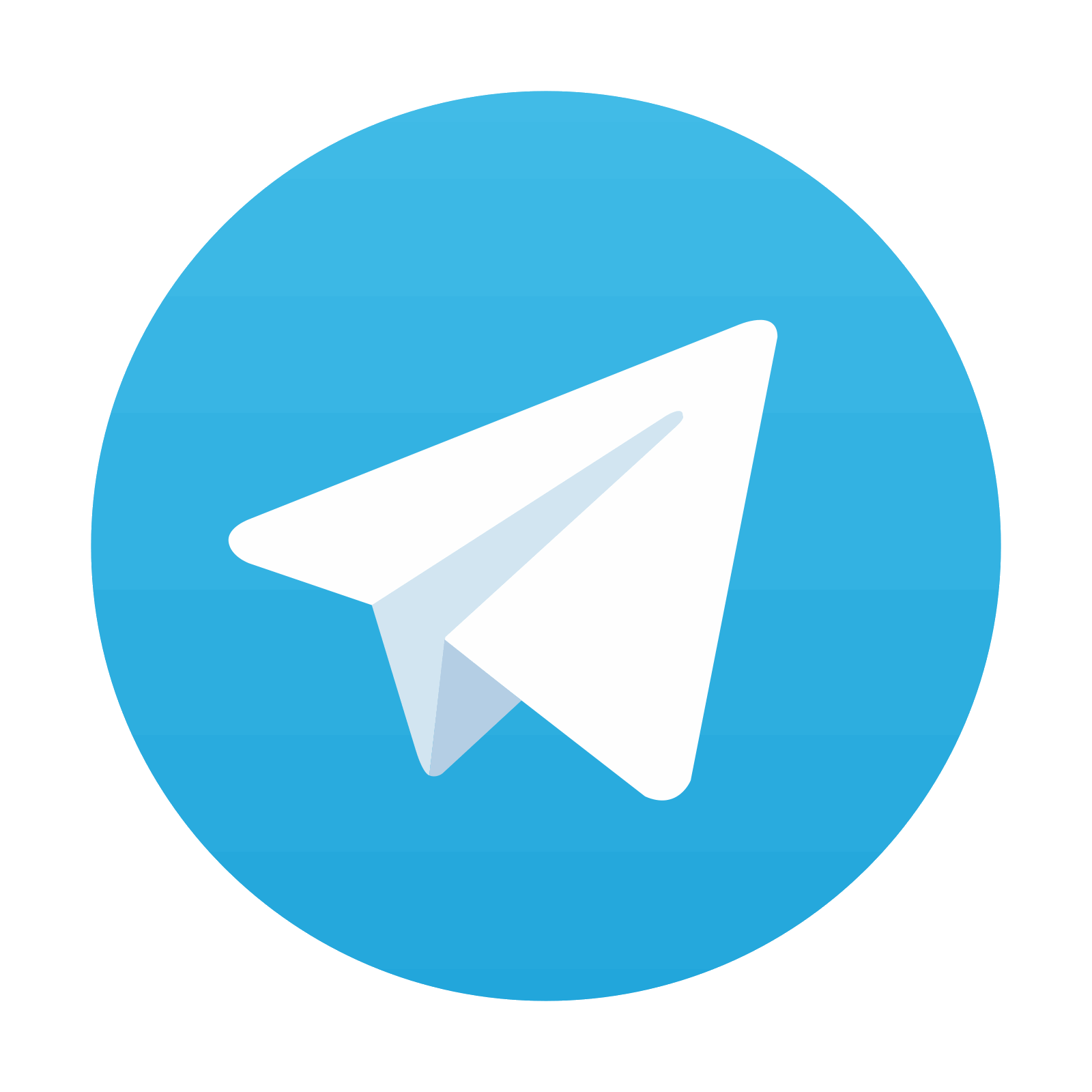
Stay updated, free articles. Join our Telegram channel
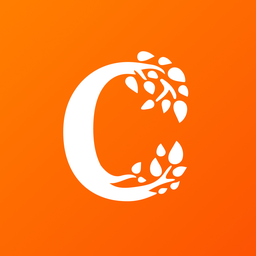
Full access? Get Clinical Tree
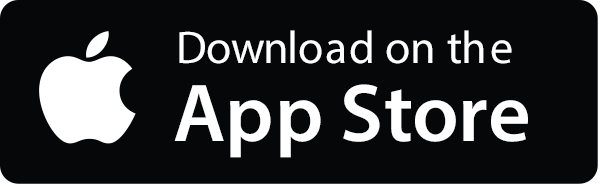
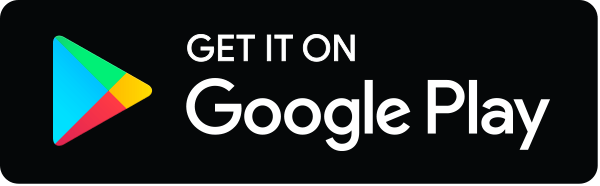