Fig. 9.1
Schematic representation of present radiotherapy workflow pathway
To date the most impactful strategy to improve the therapeutic ratio in radiotherapy has involved improvements in the accuracy and precision of radiation delivery. This has been accomplished through improved anatomic planning images (e.g., higher-resolution CT, MRI simulators) to better delineate gross disease, advancing knowledge of patterns of relapse to better define the clinical target volume (CTV) and improved immobilization and daily image-guided imaging technologies to reduce uncertainties surrounding radiation delivery. The introduction of novel imaging modalities offers an opportunity to synergistically complement these with the ability to image and target differential tumor biology.
Dose Painting
The idea of targeting tumor subvolumes based on functional imaging is often referred to as “dose painting”, a term coined by Ling and colleagues in 2005 [3]. The concept is to incorporate the quantitative output of a functional imaging modality directly into the dose prescription. This can be done as coarsely as to prescribe two tumor prescriptions, i.e., the anatomic GTV and the biologic GTV , or as finely as a prescription for each voxel scaled on the biologic parameter in question. The latter approach was first proposed by Bentzen and was coined “dose painting by numbers”, referencing the popular children’s coloring technique [4]. Needless to say, there are innumerable intermediaries between these two ends of the spectrum.
The concept of dose painting is integral to the process of exploiting personalized tumor biology with radiotherapy, either by local escalation or de-escalation to resistant or sensitive tissues, respectively. The realization of this technique requires a rigorous preliminary evaluation, including assessing each modality’s ability to quantify a relevant oncologic parameters, how said parameters are affected by treatment, and ultimately determining a clinically relevant dose–response relationship. Furthermore, the feasibility of integration of these new modalities will require careful quality assurance testing regarding the interaction with the current radiotherapy workflow.
Functional Imaging and the Basic Radiobiology
The radiobiologic principles —4 Rs—were first described by Withers as a means of explaining the tumoricidal effects of radiotherapy [5]. Withers described the differential repair, cell cycle redistribution, repopulation of tumor cells, and reoxygenation. These concepts fit well with the empirical evidence of differential cell kill noted in standard fractionated radiotherapy and observed oxygen enhancement. Intrinsic radiosensitivity was added to this framework over a decade later by Steel et al. as a means of rationalizing the differential cell kill effects based on tumor histology [6]. Over the past 30–40 years since these concepts were introduced, the understanding of cancer biology has grown exponentially, punctuated with the translational success of molecularly targeted agents in clinical care. Nonetheless, the original 4 Rs remain a fundamental pillar of radiotherapy due to their ability to explain empiric observations, with literature consistently being published to better explain the molecular underpinnings of this success [7]. As such, the 4 Rs provide a natural and useful framework in which to discuss the integration of functional imaging within the context of radiotherapy and the potential for optimizing the therapeutic ratio (Fig. 9.2). The workflow outlined in Fig. 9.1 also illustrates the potential for integration of adjunct imaging with adaptation. The following sections will describe the role of functional imaging modalities within this framework and highlight both current clinical applications and potential for future integration of ongoing translational research. We limit the discussion to the most utilized 3 Rs, omitting redistribution due to lack of dedicated imaging.
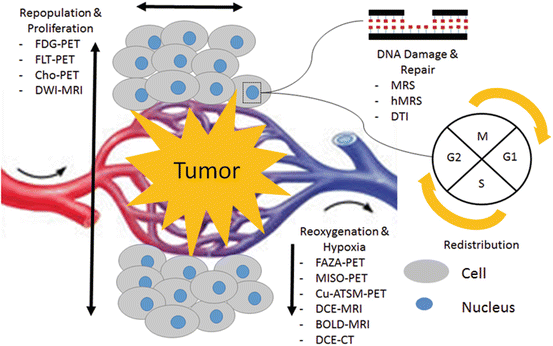
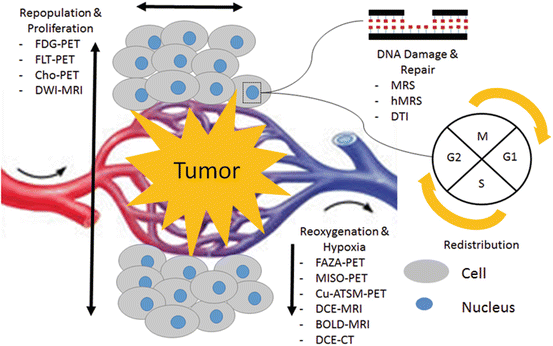
Fig. 9.2
Schematic representation of the 4 Rs of radiotherapy
Repopulation: Metabolism and Proliferation
The malignant transformation of normal tissues and the subsequent growth of new neoplasms rely on a host of molecular changes that lead to the acquisition of pro-growth capabilities, including (but not limited to) self-sufficiency in growth factors, insensitivity to antigrowth signals, limitless proliferative potential, sustained angiogenesis, and evasion of apoptosis [8]. A growing tumor is often first detected clinically (or with planned radiographic screening) as a growing mass, representing an underlying abnormal proliferation of cells. This represents the standard target of radiotherapy and is delineated in an anatomical fashion on CT or MRI. Functional imagining provides an opportunity to evaluate the underlying molecular signatures of cancer growth, to potentially aid in targeting, adaptation, and assessment of treatment response.
Glycolysis
Glycolysis is the cellular conversion of glucose to energy, a process that is upregulated in the proliferative atmosphere of malignancy. Currently, the most heavily utilized functional imaging modality in cancer care exploits this phenomenon by modifying a glucose molecule to contain a positron-emitting fluorine-18 isotope, namely, fluorodeoxyglucose (FDG) . FDG-based positron-emission tomography (PET) is able to image the differential uptake of glucose in tumor cells over neighboring normal cells owing in part to their higher metabolic requirements. The common measurement used in PET is the standard uptake value (SUV) , defined as voxel activity per dose injected per body mass. FDG-PET has been shown to be extremely powerful in identifying cancer cells, even in the absence of abnormal anatomic morphology. The combination of PET with CT has resulted in vastly improved sensitivity in detecting occult disease missed by CT, resulting in improved locoregional targeting and where appropriate omitting intensive curative treatments in patients with distant metastasis. In non-small cell lung cancer (NSCLC) (Fig. 9.3), the addition of FDG-PET to conventional staging assessment has shown a change in planned management in 20–30 % of patients, mainly in reducing the number of futile thoracotomies [9]. Similarly in esophageal cancer (Fig. 9.4), FDG-PET has been found to improve the sensitivity for detection of metastatic disease by up to 20 % over CT alone [10]. In head and neck cancers, the use of FDG-PET/CT has become common due to the high sensitivity, specificity, and accuracy for metastatic disease (>75 %) [11] and the finding that local radiotherapy plans changed from curative to palliative intent in 13 % of patients when FDG-PET information was added [12]. FDG-PET has also had a large impact in the care of lymphoma patients, particularly those with Hodgkin’s lymphoma (HL) (Fig. 9.5). Staging FDG-PET results in stage migration in ~25 % of patients with HL and a change in management in up to 15 % [13]. The standard of care for numerous malignancies now involves FDG-PET/CT in the work-up, including the lung and lymphoma to name a few.
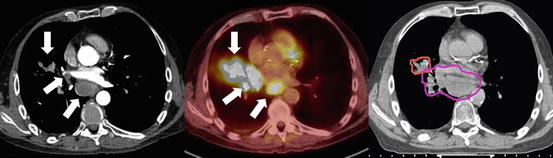
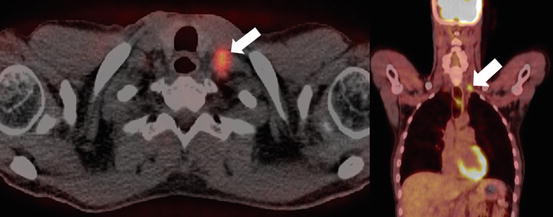
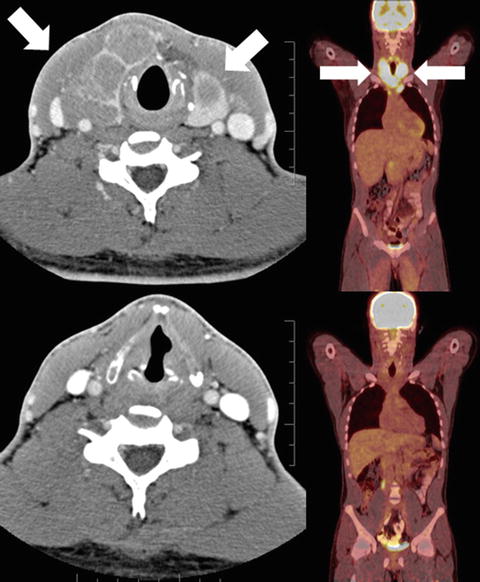
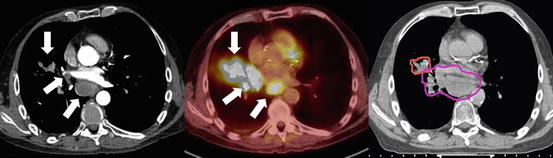
Fig. 9.3
Baseline CT (left), FDG-PET (middle), and planning CT (right) images of a patient with stage IIIA (T2N2) lung adenocarcinoma treated with concurrent chemoradiation. White arrows denote the primary tumor and nodal disease on CT and FDG-PET images; the solid lines delineate the radiotherapy targets, primary (red) and nodal (magenta) internal target volumes, respectively
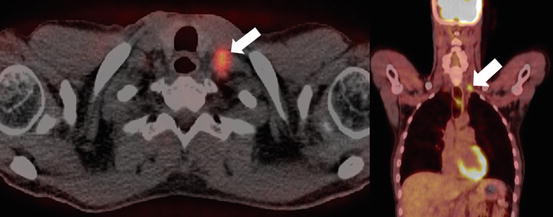
Fig. 9.4
Baseline axial and coronal FDG-PET images of a patient with squamous cell carcinoma of the upper thoracic esophagus. The FDG-PET scan identified a supraclavicular node (white arrows) that was subsequently included in the radiation treatment volume
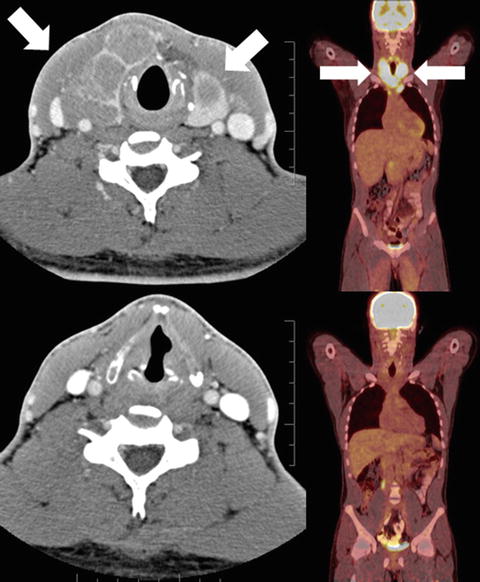
Fig. 9.5
CT (left) and FDG-PET (right) images of a patient with stage IIAX Hodgkin’s lymphoma at baseline (top) and after the planned four cycles of combination chemotherapy and involved field radiotherapy (bottom) . White arrows denote the tumor at baseline. The patient achieved complete CT and metabolic response posttreatment
The rise in utilization of FDG-PET as a staging investigation has led investigators to study its use as a quantitative biomarker via the SUV measurement. In an examination of patients from three prospective clinical trials evaluating chemoradiation in non-operable stage II and stage III NSCLC patients, baseline FDG-PET information was found to be predictive of local recurrence [14, 15]. Volumes generated by delineating the 70 % of pretreatment SUVmax were well correlated with the 90 % SUVmax recurrence volume.
Similar results have been demonstrated in cancers of the head and neck, which have shown that local recurrences are more likely to occur within FDG-avid areas and that the degree and volume of avidity is associated with both local and distant recurrence rates [16, 17]. Furthermore, analogous studies have been conducted with the addition of interim FDG-PET analysis partway through definitive head and neck radiotherapy and have demonstrated that the lack of FDG response is associated with inferior outcome [18]. Studies done in esophageal and rectal cancer are consistent, showing that the degree of metabolic response between pre- and posttreatment scans is associated with outcome [19, 20]. Adaptive PET-guided strategies have been investigated in Hodgkin’s and non-Hodgkin’s lymphoma alike (Fig. 9.6). In advanced-stage Hodgkin’s lymphoma , treatment with six cycles of BEACOPP (escalated) followed by PET-guided radiotherapy resulted in better freedom from treatment failure and less toxicity compared to eight cycles of the same chemotherapy regimen [21]. However, interim analysis of HD10 trial showed that omitting radiotherapy in patients with stage I/stage II Hodgkin’s lymphoma who attained a negative early PET scan after two cycles of chemo was associated with an increased risk of early relapse compared to standard combined modality treatment [22].
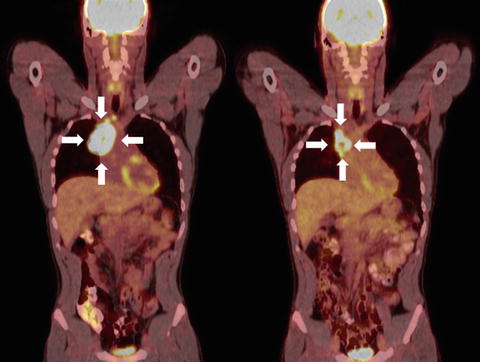
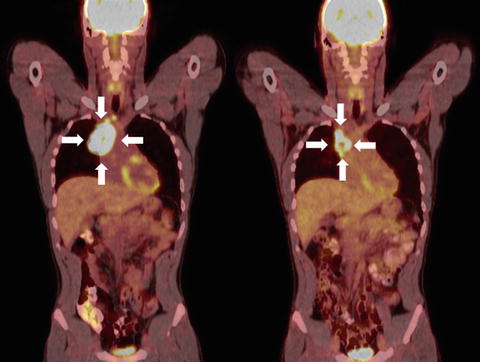
Fig. 9.6
FDG-PET scan images from baseline (left) and after six cycles of combination chemotherapy (right) of a patient with primary mediastinal diffuse large B-cell lymphoma. White arrows denote areas of metabolically active disease. This patient received 35 Gy in 20 fractions of involved field radiotherapy to the areas of persistent FDG uptake
The linkage with CT has enabled PET to easily integrate into the radiotherapy workflow, and given its promise as a biomarker, the natural extension has been to utilize the combined information directly in radiotherapy planning. The obvious benefit is to identify areas that may be missed on a standard planning CT due to normal anatomic morphology but identified via FDG avidity. Not surprisingly, numerous studies have shown the alteration of final GTV volumes with the inclusion of FDG-PET information [23–26]. FDG-PET has been shown to be the most accurate modality for delineating GTV compared to MRI and CT in pharyngolaryngeal cancer when validated against surgical specimen [27]. Studies are now being conducted to evaluate the benefit of taking this biologic information further by assessing its use as a target for dose painting. As mentioned above, studies have suggested that local glycolytic activity may correlate with resistant disease and therefore a target for dose escalation. Early dose painting studies have established the feasibility of this technique for numerous sites, including the head and neck, lung, and esophagus. Madani et al. showed the safety of dose painting by numbers in a phase I study evaluating the head and neck (non-nasopharynx ) of patients undergoing definitive radiotherapy [28]. They showed that a median CTV dose of 80.9 Gy was safely achievable. Similarly, Yu et al. demonstrated a sub-GTV volume based on the pretreatment SUV50%Max in patients with an SCC of the esophagus could be safely escalated to 70 Gy using a simultaneous integrated boost [29]. In NSCLC, studies integrating FDG-PET into the RT planning process have shown that one can modulate the OAR to CTV dose ratio using metabolic guidance to either shrink boost volumes while keeping overall integral target doses constant (resulting in lower surrounding OAR doses) or conversely by maintaining an iso-OAR dose and dose painting nearby target subvolumes. Van Der Wel et al. showed that when using a prescription dose of 60 Gy, an FDG-PET-based plan could be implemented to reduce the mean esophagus dose from 29.8 to 23.7 Gy and the average lung dose by 2 %. Conversely, for an iso-OAR dose, the mean target dose could be increased to 71 Gy [30]. A phase II study by the Belderbos group showed that the utilization of FDG-PET in planning could be used to safely boost the mean target dose to 77 Gy [31]. Table 9.1 highlights several studies investigating the integration of FDG-PET into the radiotherapy process . For a more dedicated review, please refer to excellent summaries provided by Jelercic and Shi, respectively [23, 32]. Prospective studies are now being developed to test whether these modifications can result in meaningful clinical endpoints, including RTOG-1106 investigating whether FDG-PET-guided radiotherapy can improve local control in stage III NSCLC (RTOG-1106).
Table 9.1
Summary of select studies utilizing FDG-PET in tumors treated with radiotherapy
Reference | Study type | Tumor site | N | Findings |
---|---|---|---|---|
Calais et al. [14] | Prospective | NSCLC | 39 | 70 % SUVmax-threshold subvolumes at baseline correlate with 90 % SUVmax-threshold volume at relapse (>0.51 common volume/recurrent volume) |
Bradley et al. [15] | Prospective | NSCLC | 26 | Integration of FDG-PET resulted in a GTV contour change in 58 % of patients, in 12 % the volume decreased due to improved ability to delineate in atelectatic lung, and 46 % the volume increased due to additional tumor detected |
Roh et al. [16] | Retrospective | Hypopharynx | 78 | Increased metabolic tumor volume (delineated as isocontour of SUV >2.5) was associated with inferior 4-year overall survival (HR 2.66, p = 0.002) |
Due et al. [17] | Retrospective | HNSCC | 520 | Most recurrences occur within FDG-avid areas. Recurrence density increased with % SUV isocontour delineation and was highest for 90 % SUVmax (p = 0.036) |
Kilic et al. [24] | Retrospective | Rectum | 20 | CTV targets derived with FDG-PET/CT were significantly larger than CT alone (median CTV volume 559.73 mm3 vs. 468.43 cm3, P = 0.043) |
Nkhali et al. [26] | Retrospective (planning study) | Esophagus | 10 | The median GTV volume delineated at baseline and again after 37.8–42 Gy (+ concurrent cisplatin) using FDG-PET decreased from 12.9 to 5.0 cm3 (p = 0.01), resulting in a significant reduction in the lung V20 (26.8–23.2 %, p = 0.006) |
Madani et al. [28] | Prospective | HNSCC | 39 | Phase I study showing FDG-based dose painting by numbers allowed dose escalation to a median 80.9 Gy, with no acute grade 3 toxicity and only one patient with grade 2 toxicity (mucosal ulceration). One-year local control was 87 % |
Yu et al. [29] | Prospective | Esophagus | 25 | Dose escalation to a subvolume (50 % SUVmax isocontour) to 70 Gy in 2.8 Gy fractions using simultaneous integrated boost, and concurrent chemotherapy was achievable with two grade 3 toxicities (one myelosuppression, one nausea/vomiting). One-year local control was 77.4 % |
van Elmpt et al. [31] | Prospective | NSCLC | 20 | Dose escalation to a metabolic tumor volume (50 % SUVmax) was achievable to ≥72 Gy in 15/20 (75 %) patients while maintaining planning OAR constraints |
In addition to the value of metabolic imaging at baseline, the ability to compare glycolytic activity following treatment has been shown to offer independent prognostic information. In cancers of the head and neck, FDG-PET acquired 24-week posttreatment demonstrated >90 % sensitivity and specificity in locating recurrent disease and offered an 85 % rate of concurrent targeted biopsies [33]. In locally advanced esophageal cancers , Kauppi et al. demonstrated that percentage change in SUV of the primary tumor (pre- and post-neoadjuvant chemotherapy) was independently predictive of overall survival (HR 0.966 per 1 % increase, p < 0.0001) [34]. FDG-PET response has been correlated pathologically by Bahace et al., investigating patients with superior sulcus tumors who underwent trimodality therapy and demonstrating that the maximum SUV obtained following chemoradiotherapy was correlated with the amount of remaining viable tumor cells (R = 0.55, p = 0.007) [35]. In squamous cell carcinomas of the cervix , Schwarz et al. showed that FDG-PET images acquired 3 months post chemoradiotherapy were predictive of the response. Twenty-three percent of patients with complete metabolic response experienced treatment failure, compared to 100 % of those with progressive metabolic activity and 65 % of those with partial metabolic response [36]. These results emphasize the role of FDG-PET in oncology, and particularly radiotherapy , further accentuating the importance of prospective studies integrating this information into treatment planning as discussed above.
Proliferation
The ability of tumor cells to divide and grow in a self-sustained fashion is one of the hallmarks of cancer; it has been shown that the rate of proliferation is prognostic in many cancers in many sites [37–40]. Additionally, the rate of tumor growth can increase after treatment (chemotherapy or radiotherapy), a phenomenon named “accelerated repopulation” [41]. Numerous disease sites have demonstrated a reduced rate of local control with prolonged treatment courses, highlighting the importance of this effect [42–46]. The ability to identify a tumor’s specific proliferative potential at the outset of treatment and then follow dynamic changes during/after treatment may be beneficial in modern radiotherapy.
PET
The functional imaging tracer, 3′-deoxy-3′-18F-fluorothymidine (FLT) , has been proposed as a noninvasive imaging biomarker for proliferation based on its biochemical pathway in dividing cells. FLT is taken up by cells and is phosphorylated by thymidine kinase-1 (TK1) , trapping it within the cell (Fig. 9.7). TK1 is upregulated in S-phase and is thus an indirect measurement of cell division. FLT uptake has been shown to correlate with the gold standard in pathologic proliferative status, Ki67 , in a number of cancer sites, and in and of itself has shown to be a prognostic biomarker based on treatment response [47–49]. In squamous cell cancers of the head and neck, a decrease in FLT uptake (SUVmax and PET-segmented GTV) during the first 2 weeks of definitive radiotherapy (± chemotherapy) was associated with better 3-year disease-free survival (88 % vs. 63 %, P = 0.035, and 91 % vs. 65 %, P = 0.037, respectively) [50]. The use of FLT imaging has been extensively investigated in terms of targeted growth signal modifiers, most commonly modulators of the epidermal growth factor receptor (EGFR) . One study showed that early changes in FLT-PET during the first week erlotinib treatment predicted significantly longer progression-free survival (HR 0.3) in patients with NSCLC [51]. Furthermore, FLT has been shown to be a better marker of disease response than FDG-PET during radical chemoradiation , although the results are still pending on if this will translate to a difference in patient outcome in a prospective setting [52].
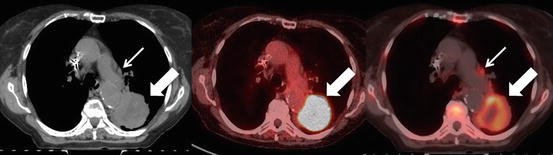
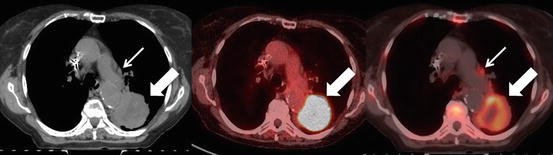
Fig. 9.7
Baseline CT (left), FDG-PET (middle), and FLT-PET (right) images of a patient with newly diagnosed non-small cell lung cancer. The thick white arrow indicates the primary tumor. The thin white arrow represents a mediastinal lymph node that is avid on FLT-PET scan, but not on the corresponding FDG-PET scan (courtesy of Dr. Meredith Giuliani)
Similar to FDG-PET, FLT-PET is commonly integrated with CT scanning, providing a relatively straightforward integration into the radiotherapy pathway. Planning studies investigating this integration have provided promising preliminary results. In thoracic esophageal cancers, the GTV volumes constructed using FLT-based parameters were generally 10 % smaller than anatomic delineations, resulting in a 10 % reduction in mean lung and heart doses (p < 0.01) and showed higher correlation with final pathologic size [53]. Planning studies have also shown the feasibility of boosting areas of high proliferation in tumors of the oropharynx and rectum [54, 55]. A comprehensive review focused on FLT in oncology is provided by Tehrani et al. [56].
Choline is a substrate for synthesis of phosphatidylcholine, which is a major component of the cellular membrane. Choline can be tagged with a carbon-11 or fluorine-18 positron-emitting radionuclide for use in PET scanning (Cho-PET). With cellular turnover elevated in malignancies, accumulation of choline can be demonstrated in areas of active disease. Cho-PET has been most heavily investigated in prostate cancer and has shown improved sensitivity and specificity for both regional and distant disease detection over conventional staging modalities [57]. Cho-PET has also been investigated in regard to identifying dominant tumor nodules within an intact prostate, possibly for local dose escalation strategies. Currently the standard imaging for this task is multi-parametric MRI (mpMRI). However, a meta-analysis by Chan et al. showed that while Cho-PET alone was roughly equivalent to mpMRI in identifying a dominant nodule, the combination of both had improved sensitivity and specificity over either alone [58]. Planning studies using Cho-PET for defining subvolumes for dose escalation up to 90 Gy have shown gains in estimated tumor control probability (TCP) without exceeding OAR tolerances [59, 60]. Pinkawa et al. implemented this technique up to 80 Gy and showed no adverse effects on toxicity or quality of life [61]. The utilization of Cho-PET has been more widely accepted in the setting of recurrent disease and now represents the standard of care in many countries, including the United States, for restaging prior to definitive salvage treatment [62]. There is single institution data supporting the feasibility of Cho-PET-directed salvage treatment up to 74 Gy; however larger series and longer-term outcomes are needed [63]. Table 9.2 provides a summary of relevant literature pertaining to radiotherapy and proliferative imaging modalities.
Table 9.2
Summary of select studies utilizing FLT and choline PET in tumors treated with radiotherapy
Reference | Tracer | Study type | Tumor site | N | Findings |
---|---|---|---|---|---|
Hoeben et al. [50] | FLT | Retrospective | HNSCC | 48 | A relative change in tumor SUVmax ≥ 45 % from baseline to after 1.5 weeks of treatment (median 14 Gy, range 10–24 Gy) was associated with improved disease-free survival (88 % vs. 63 %, p = 0.035) |
Everitt et al. [52] | FLT | Prospective | NSCLC | 20 | Visual analysis of both FLT and FDG PET after 2 weeks of CRT (compared to baseline) showed that 52 % of patients had a partial metabolic response, while 76 % had a partial proliferative response, suggesting earlier tumor cell proliferation is affected more rapidly than metabolism |
Troost et al. [55] | FLT | Prospective | HNSCC | 10 | A significant decrease in SUVmax was seen during radiotherapy (compared to baseline), greatest after 2 weeks of treatment (20 Gy), but continuing through 4 (40 Gy) weeks of treatment (7.6 vs. 3.1 vs. 1.7, p = 0.0001 and 0.001, respectively) |
Chang et al. [59] | Cho | Prospective (planning study) | Prostate | 8 | A SIB to 90 Gy to a subvolume defined by 70 % SUVmax threshold resulted in a higher TCP across all patients (mean increase 37 %, p < 0.001) with no significant change in NTCP rectum and bladder |
Pinkawa et al. [61] | Cho | Prospective | Prostate | 67 | Delivery of a SIB to 80 Gy to a subvolume defined by a tumor-to-background ratio >2 did not result in significantly increased acute or late bladder/bowel toxicity or decreased QoL (median 2 and 19 months post-RT) compared to a similar cohort not treated with SIB |
Diffusion-Weighted MRI
MR diffusion imaging is an imaging sequence based on the thermally driven random motion of water molecules (Brownian motion). By applying strong gradient pulses, local diffusivity can be measured, and regions can vary depending on the local microenvironment. Generally, higher cellular density equates to lower diffusivity [64]. The metric generally used to quantify this diffusivity within a given volume element is referred to as the apparent diffusion coefficient (ADC) , given in units of area/time. The term “apparent” is used because true diffusion is affected by innumerable variables which cannot be individually quantified in-situ (i.e., intravoxel motion, microvascular perfusion, physical obstacles within the cell, etc). Lower ADC values represent more restricted diffusion (Fig. 9.8); it has been reported across numerous sites that regions of malignancy have lower ADC values than surrounding healthy tissue [65–68]. Intuitively, regions of increased proliferation lead to areas of increased cellularity; and ADC has also been shown to correlate with Ki67 in both bladder and breast cancer [69, 70]. Furthermore, areas of reduced ADC have been shown to be associated with higher grades of breast, bladder, endometrial, and prostate cancer [69, 71–73]. The use of DWI in the identification of high-grade prostate cancer has been particularly useful and relevant for management decisions and now features prominently in the prostate imaging reporting and data system (PI-RADS) classification system for MRI evaluation of prostate cancer [74]. In a recent review by Futterer et al., DWI was able to provide a negative predictive value of up to 98 % for the presence of greater than Gleason 6 prostate cancer [75]. Baseline ADC values have also been shown to be biomarkers of disease-free survival in patients undergoing radical chemoradiation for squamous cell carcinomas of the cervix. A retrospective study by Micco et al. demonstrated that a higher pretreatment mean tumor ADC was associated with better disease-free and overall survival in patients treated with chemoradiation on univariate analysis (HR 0.56, p = 0.007; HR 0.46, p = 0.02) [76]. Similar findings were reported by other groups investigating cervical cancer [77, 78]. In postoperative recurrences of cervical cancer , a tumor ADC >0.95 × 10−3 mm2/s was predictive of a lack of complete response to chemoradiation in bulky lesions (sensitivity 85.7 %; specificity 100 %, p = 0.05) [79]. In rectal cancer , a systemic review showed that pretreatment ADC was predictive of pCR following neoadjuvant chemoradiation with a negative predictive value of 90 % and a specificity of 68 % [80].
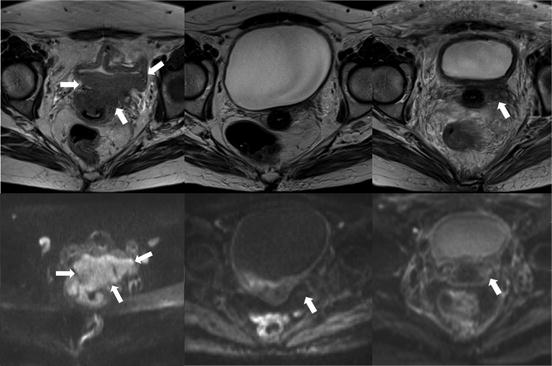
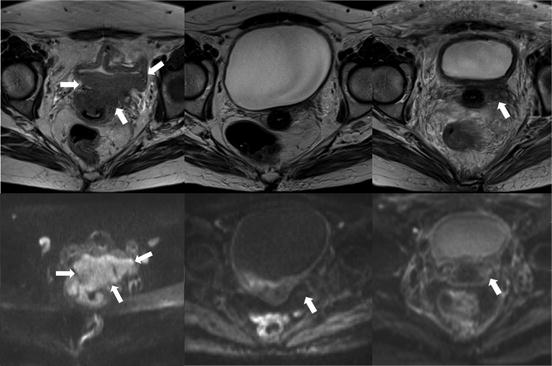
Fig. 9.8
Axial MRI images acquired pretreatment (left), 3 months after completion of chemoradiation (mid) and at the time of recurrence 1 year post-chemoradiation (right) in a woman who presented with stage IVA cervical squamous cell carcinoma. Along the top are T2-weighted images, and below are the corresponding diffusion-weighted images (b = 800 s/mm2). White arrows denote initial disease and the area of recurrence. Note the persistent restricted diffusion even at time of radiographic complete response posttreatment
Much like FLT imaging, DWI-MRI has shown promise in measuring the dynamic changes of malignancies throughout and after treatment, offering an in vivo barometer of treatment effect. The hypothesis is that treatment-induced cell death will result in lower cellular density, reducing the restrictions to intercellular water diffusion and therefore increasing local ADC. Unlike single pretreatment measurements, increasing ∆ADC with treatment (ADCpost–ADCpre) has consistently shown positive associations with outcome. A work by Yu et al. demonstrated that patients treated with stereotactic radiotherapy for hepatocellular carcinoma with an increment of ≥20 % in ADC value (6 months vs. pretreatment) had significantly better local progression-free survival at 1 year [81]. Greater increases in tumor ADC early (mostly 1–3 weeks) during RT ± concurrent chemotherapy have been associated with better response in many cancers: high-grade glioma [82], head and neck squamous cell carcinoma [83], nasopharynx cancer [84], liver tumor (hepatocellular carcinoma, liver metastases, cholangiocarcinoma) [85], rectal cancer [86–88], and cervix cancer [89–91]. In particular, a meta-analysis done by Fu et al. demonstrated a significant association between ∆ADC and the status of residual tumor post-chemoradiation in locally advanced cervical cancer patients (p < 0.001), suggesting its utility in monitoring treatment response [92]. There is currently limited work investigating the real-time integration of DWI-MRI directly into radiotherapy planning, but preliminary work does show promise [93–95]. Table 9.3 provides a representative summary of evidence investigating DWI-MRI in conjunction with radiotherapy.
Table 9.3
Representative summary of literature investigating DWI-MRI utility in radiotherapy
Reference | Study type | Tumor site | Timing of DWI | N | Findings |
---|---|---|---|---|---|
Micco et al. [76] | Retrospective | Cervix | Pretreatment | 49 | Decreased baseline mean tumor ADC was associated with inferior DFS and OS on univariate analysis (HR 0.56, p = 0.007, and HR 0.46, p = 0.02, respectively) |
Nakamura et al. [77] | Retrospective | Cervix | Pretreatment | 80 | Decreased baseline mean tumor ADC was associated with inferior DFS on multivariate analysis (HR 4.11, p = 0.013) |
Chopra et al. [79] | Prospective | Cervix (recurrent) | Pretreatment | 20 | Mean tumor ADC >1.0 × 10−3 mm2/s was predictive of partial response to CRT (sensitivity 85.7 %; specificity 100 %, AUC = 0.96, p = 0.05). Of nine partial responders, three had residual disease spatially corresponding to baseline area of restricted diffusion |
Joye et al. [80] | Meta-analysis | Rectal | Pre- and posttreatment | 226 | A decreased baseline tumor ADC and increased ∆ADC (pre–post was predictive of pCR following neoadjuvant chemoradiation-NPV 90 %, PPV 35 %; and NPV 94 %, PPV 46 %, respectively) |
Yu et al. [81] | Retrospective | HCC | Pre- and posttreatment | 48 | ∆ADC [(pre–post)/pre] >20 % was associated with better 1-year local control (100 % vs. 75.8 %, p = 0.02). Post-treatment scans were taken 3–5 months following SBRT (15–20 Gy/3 fractions) |
Hamstra et al. [82] | Prospective | High-grade glioma | Pre-, mid-, and posttreatment | 60 | Patients with an increase in ADC in >4.7 % of voxels within the GTV after 3 weeks of RT (30 ± 4 Gy) had longer median survival (52.6 months vs. 10.9 months, p < 0.003) |
Galban et al. [83] | Prospective | HNSCC | Pre- and mid-treatment | 15 | The mean whole tumor ADC after 3 weeks of CRT was significantly higher in patients with radiographic CR than in those with PR (0.0016 mm2/s vs. 0.0014 mm2/s, p < 0.05) |
Chen et al. [84] | Prospective | Nasopharynx | Pre-, mid-, and posttreatment | 31 | ∆ADC (mid-pre and post-pre) of both primary tumor and metastatic lymph nodes was significantly higher in patients with CR than those with residual disease (p ≤ 0.05) |
Fu et al. [92] | Meta-analysis | Cervix | Pre- and posttreatment | 577 | Post-treatment ADC was significantly higher than pretreatment ADC in patients treated with CRT (standardized mean difference 2.95 mm2/s, 95 % CI 2.19–3.72, p < 0.001) |
Summary
A trait common to many malignancies is the increase in cellular proliferation , which can manifest as local increases in glycolysis and cellular density. Novel functional imaging modalities have probed each of these facets directly, including quantifying FDG uptake with PET, assessing cellular density with DWI-MRI , and directly estimating local proliferation rates with FLT-PET . Each of these modalities has been shown to be useful in oncologic imaging and have been investigated to improve staging. Each is increasingly showing promise as a quantitative peri-treatment biomarker for treatment response and/or long-term outcome. Retrospective studies have shown benefit with integration into radiotherapy planning/dose painting, and larger prospective trials are now ongoing.
Reoxygenation: Hypoxia and Vascularization
Hypoxia refers to the phenomenon where cells are deprived of oxygen, which is essential for a variety of cellular functions. A prolonged hypoxic environment leads to changes in cellular metabolism and genomic expression that can result in alterations of glycolysis and DNA repair. Tumor hypoxia is often a result of the rapid proliferation and abnormal vasculature associated with malignant growth. The chaotic and unorganized growth of cancer creates an unpredictable variation of oxygenation among and within tumors. Hypoxic tumors are more resistant to treatment and are associated with poor clinical outcome [96, 97]. There is strong prospective evidence that modification of hypoxia itself leads to improved tumor control [98–100]. With regard to radiotherapy, in particular, the lack of oxygen disrupts one of the major radiobiologic methods of cell killing —free radicals. The deposition of high-energy photons leads to local ionization; the resultant free radicals directly inflict DNA damage. This damage can become permanent with the fixation of nearby oxygen to become stable organic peroxides. In the absence of oxygen, this damage can be repaired more effectively. The gold standard for measuring hypoxia is polarographic electrodes, which can measure oxygen tension directly in the tissue [101, 102]. However, this method requires the invasive introduction of an electrode into the tissue itself; therefore, it is not feasible in many cancer sites. The ability to detect hypoxia noninvasively by imaging enables not only better quantification of tumor heterogeneity but also a potential biologic target for radiotherapy.
PET
A number of positron-emitting tracers have been evaluated for imaging hypoxia. The most prominent probes are nitroimidazole-based, a compound that was first shown by Chapman to remain trapped within hypoxic cells [103]. Among the nitroimidazole-based probes , the most investigated are fluoromisonidazole (FMISO) , diacetyl-bis-N4-methylthiosemicarbazone (Cu60 or Cu64-ATSM), and fluoroazomycin arabinoside (FAZA) (Fig. 9.9). These probes have shown promise as not only a reliable biomarker of hypoxia but also an association with outcome across many tumor types. Pre-treatment PET imaging of tumor hypoxia in patients with head and neck, lung, brain, and cervical cancers has been shown to correlate with disease-/progression-free survival [104–107], cancer-specific survival [108, 109], and overall survival [110–113] following (chemo)radiation. A meta-analysis by Horsman across multiple tumor types and a variety of tracers showed that hypoxic tumors were more likely to have poor response to radiotherapy (OR 0.27, 95 % CI, 0.18–0.39) [114].
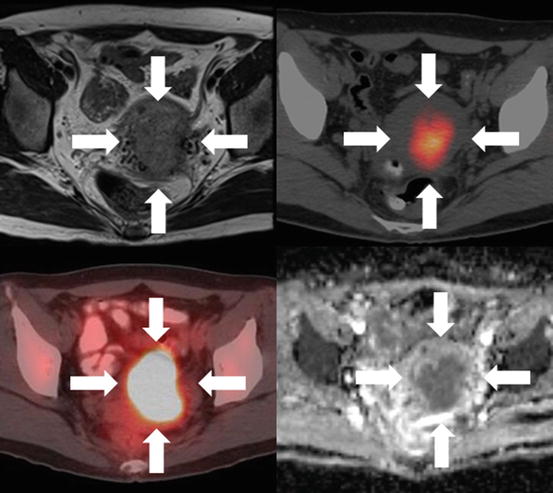
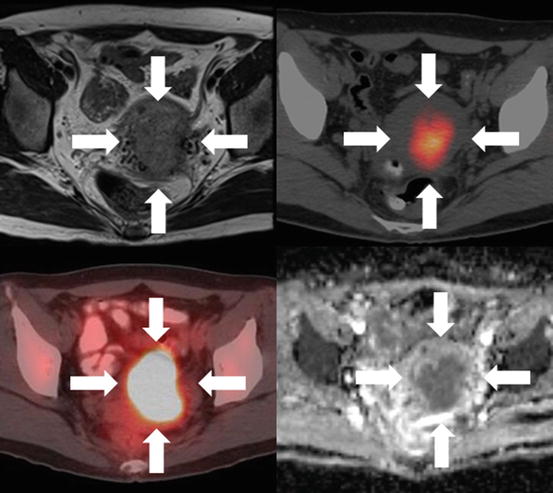
Fig. 9.9
Baseline axial T2 MRI (left upper), FAZA-PET/CT (right upper), FDG-PET (left lower), and ADC map (right lower) images of a patient with stage IIIB cervix squamous cell carcinoma. White arrows indicate the primary tumor. Note the tumor shows focal FAZA uptake, strong FDG uptake, and restricted diffusion
Although a meta-analysis showed that hypoxia-modifying therapies improved locoregional control (HR 0.77; 95 % CI 0.71–0.86) and overall survival (HR 0.87; 95 % CI 0.80–0.95), they have not been adopted in clinical practice because of practical limitations and toxicities [100]. Initial studies looked at ameliorating the hypoxic environment directly via improving oxygenation (carbogen + nicotinamide), hypoxic radiosensitizers (e.g., nimorazole), or direct hypoxic cytotoxins (e.g., tirapazamine). These strategies are not specific to the spatial distribution of hypoxia. Radiotherapy allows for integrated and personalized hypoxia targeting via dose painting. Several dosimetric studies highlighted the feasibility of this approach without excess dose to the OAR. Planning studies in carcinomas of the head and neck using FAZA-PET have shown the ability to dose escalate hypoxic regions in a simultaneous boost up to 86 Gy, without significant effect on existing OAR dose constraints. Specifically, Servagi-Vernat et al. showed that this adaptive FAZA-based dose painting is feasible with FAZA-PET imaging at three time points (baseline, post 7 and 12 fractions), offering the ability to target hypoxic regions dynamically over time [115]. Similarly, dose escalation to hypoxic regions identified by FMISO-PET beyond 80 Gy is feasible without excessive increase of dose to the OARs [116, 117].
An important limitation of dose painting based on hypoxic PET signals is the temporal instability of hypoxia. Radiotherapy based on pretreatment scans inherently assumes a robust target throughout treatment. Various studies have suggested that this may not be the case in regard to hypoxia, and specifically both acute and chronic hypoxia can be important in radiation resistance. Work by Lin et al. investigated this prospectively in a group of head and neck cancer patients who had consecutive pre-treatment FMISO-PET scans 3 days apart. They found that the mean D95 for the hypoxic GTV was an average of 7 Gy lower (range 3–12 Gy) when the initial plan was applied to the second scan [118]. Bollineni et al. performed serial FAZA-PET imaging in patients with HNSCC and NSCLC and found four cases: (1) increasing hypoxia, (2) decreasing hypoxia, (3) stable hypoxia, and (4) stable non-hypoxia with patients falling into any one of these categories [119]. Further work on the reproducibility, temporal variability, and adapting radiotherapy to hypoxic subvolumes identified by hypoxia tracers is required.
In addition to its prognostic value, PET imaging with FAZA can also monitor and/or predict response to hypoxia-targeted therapy. Xenograft studies showed that FAZA tumor uptake declined by 55–70 % 1–3 days after administration of BAY 87-2243, a preclinical inhibitor of mitochondrial complex I that decreases tumor hypoxia and sensitizes tumor to radiation [106, 120]. Another xenograft study showed FAZA uptake to predict response to hypoxic cytotoxin tirapazamine and radiation [121]. Table 9.4 provides a representative review of the literature of PET-based hypoxia imaging in conjunction with radiotherapy, and further description of the use of PET-based hypoxia imaging can be found in excellent reviews by Padhani and Fleming [122, 123].
Table 9.4
Summary of select PET-based hypoxia imaging studies in tumors treated with radiotherapy
Reference | Tracer | Study type | Tumor site | N | Findings |
---|---|---|---|---|---|
Mortensen et al. [104] | FAZA | Prospective | HNSCC | 40 | Significant difference in 2-year DFS between patients with hypoxic tumor at baseline (T/M ≥1.4) vs. non-hypoxic tumor (60 % vs. 93 %, respectively) |
Zips et al. [107] | FMISO | Prospective | HNSCC | 25 | Greater hypoxic volume and tumor-to-background ratio after 1 and 2 weeks of RT were associated with inferior local PFS |
Dehdashti et al. [108] | Cu60-ATSM | Prospective | Cervix | 38 | Significant difference in 3-year PFS between patients with hypoxic tumor (T/M > 3.5) vs. normoxic tumor (28 % vs. 71 %, respectively) |
Kikuchi et al. [109] | FMISO | Prospective | HNSCC | 17 | Patients with hypoxic tumors (SUVmax ≥ 2.3 or T/M ≥ 1.3) pre-RT had lower local control rates |
Spence et al. [113] | FMISO | Prospective | GBM | 22 | Increasing hypoxic subvolumes of tumor (number of voxels with T/B > 1.2) and maximum T/B value were each independently associated with inferior OS following CRT (log HR 0.05; 1.57, respectively, p < 0.05) |
Servagi-Vernat et al. [115] | FAZA | Retrospective (planning study) | HNSCC | 12 | Hypoxic subvolumes within the GTV (SUVtumor >3σ * SUVmuscle) could be safely boosted to 86 Gy without violating OAR tolerances |
Chang et al. [116] | FMISO | Retrospective (planning study) | HNSCC | 8 | A mean increase in TCP of 20 % could be achieved by targeting hypoxic subvolumes (T/M > 1.5) to 84 Gy (compared to 70 Gy plan). The 84 Gy plan increased the mean parotid and mandible NTCP by 18 % and 25 %, respectively, although the differences were not statistically significant |
Henriques de Figueiredo et al. [117] | FMISO | Retrospective (planning study) | HNSCC | 10 | Dose escalation to a hypoxic target volume (automatic segmentation based on adaptive Bayesian method) (79.8 Gy from 70 Gy standard plan) led to a mean increase in TCP of 18.1 % with a mean increase in parotid NTCP of 4.6 %
![]() Stay updated, free articles. Join our Telegram channel![]() Full access? Get Clinical Tree![]() ![]() ![]() |