Tissue
Reference
Methodology demonstration of differentiation self-renewal
In situ localization by donor cell marker
In situ localization by functional marker
In vitro explant
Demonstration in vivo
Hematopoietic stem cell
+
+
+
+
Endothelial cell (blood vessel)
+
+
+
−
Liver (hepatocyte, oval cell, biliary duct cell)
+
+
+
−
Esophagus
+
+
+
+
Lung
+
+
+
−
Skeletal muscle
+
+
+
−
Cardiac muscle
Yoon et al. (2005)
+
+
+
−
Marrow stromal cell
Anklesaria et al. (1989)
+
+
+
+
Osteoblast
+
+
+
−
Chondrocyte
Zhou et al. (2005)
+
+
+
−
Pancreas
+
+
+
−
Intestine
Ishikawa et al. (2004)
+
+
+
−
Skin (dermis)
Deng et al. (2005)
+
+
+
−
Central nervous system neurological cell
+
+
+
−
Retina
+
+
+
−
Peripheral nervous system neurons, spinal cord
+
+
+
−
Ear
Li et al. (2003)
+
+
+
−
Germ cell
Lue et al. (2007)
+
+
+
−
Breast
+
+
+
−
Salivary gland
Lombaert et al. (2008)
+
−
+
−
Bone marrow transplantation can result in epithelial organ recovery, but this need not be attributed to transplanted stem cells (Fig. 1 ). The stem cell is not the same as committed progenitor cells. Bone marrow committed progenitor cells can migrate into an irradiated tissue, proliferate, and assume characteristics of that tissue either through the inductive microenvironment causing differentiation, or through cell fusion (Ogle et al. 2005). However, stabilization of tissue damage and transient early appearance of donor cell-derived marked cells within tissue does not prove that bone marrow-derived stem cells were responsible.
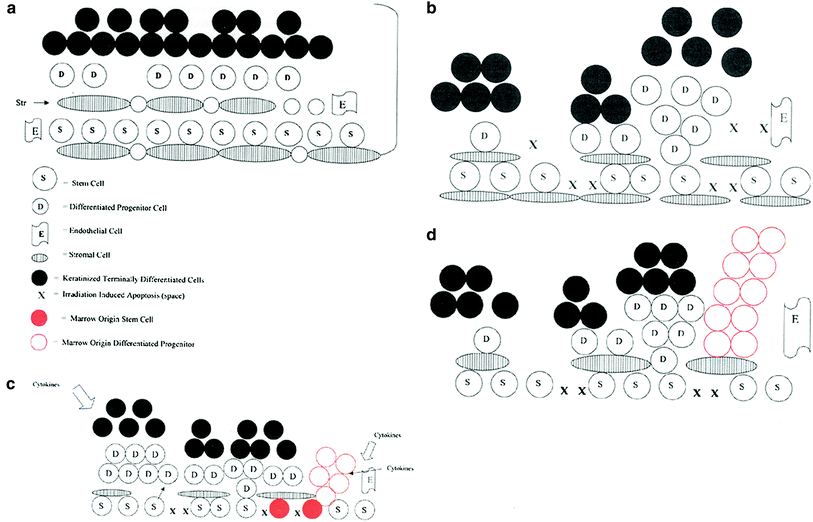
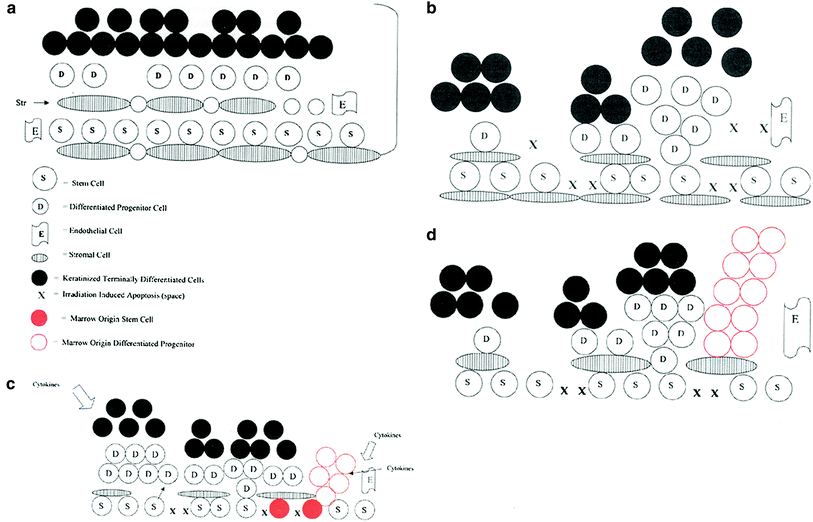
Fig. 1
Irradiation-induced bone marrow stem cell migration to epithelial tissues via the circulation serves as a restorative countermeasure to stimulate organ recovery. a Esophagus stem cells and stromal cells in a basal state. b Esophagus irradiation depletes basal stem cells causing proliferative response. c The effect of bone marrow stem cell regenerative migration (red) into the esophagus restoring the epithelial stem cell niche. d The effect of differentiated/committed bone marrow-derived cells (not true stem cells) moving into the irradiated esophagus restoring committed esophageal progenitor cells (red). True esophageal stem cells remain in their niche and repopulate the esophagus at a later time pushing out the marrow origin cells (Greenberger and Epperly 2009, reproduced with permission of the publisher (Elsevier, Inc.).)
The true definition of a stem cell is that it retains capacity for not only differentiation but self-renewal to generate other stem cells. Studies in which self-renewal has been documented represent a small subset of the total published studies reporting bone marrow stem cells derived regeneration of epithelial organs (Table 1). It is well established that damage to the microenvironment of the irradiated organ can limit bone marrow-derived stem cell or differentiated cell homing and proliferative capacity in that organ (Fig. 2) (Quesenberry et al. 2005; Epperly et al. 2004; Niu et al. 2008).
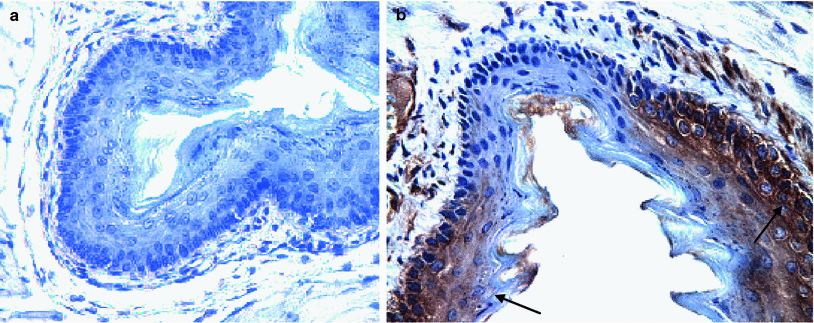
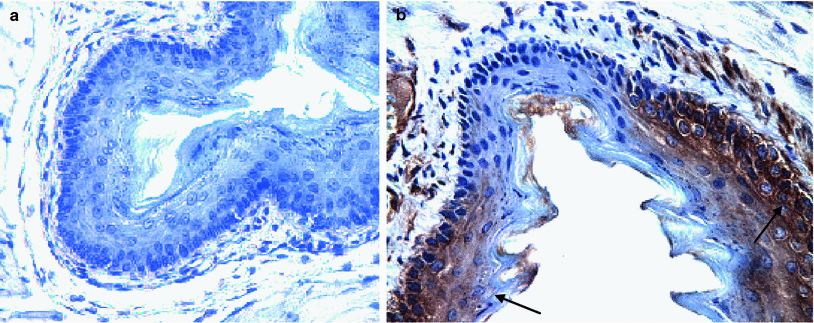
Fig. 2
ROSA positive male donor bone marrow-derived squamous epithelium in MnSOD-PL treated irradiated esophagus of C57BL/6HNsd female mouse. LacZ expression in ROSA positive cells (brown color arrows). Female C57BL/6NHsd mice were administered MnSOD-PL (100 μg plasmid DNA in 100 μl lipofectin) at the top of the esophagus and the mice were permitted to swallow. 24 h later mice were irradiated to 27 Gy to the upper body. Five days later mice were injected with 1 × 106 bone marrow cells from a ROSA (LacZ+) male mouse. Mice were sacrificed 14 days later, esophagus removed, fixed in 10 % formalin, sectioned, and stained for LacZ expression using goat anti-LacZ antibody followed by biotinylated labeled antigoat antibody and ExtrAvidin-Peroxidase reagent (Sigma Chemical Co., St. Louis, MO). Nuclei were stained with hematoxylin. a Esophagus section from an MnSOD-PL treated, irradiated control mouse. b Section from a mouse receiving MnSOD-PL before irradiation and injected ROSA bone marrow. LacZ positive foci of ROSA bone marrow (arrows) (b) (×40)
Damage to the irradiated tissue microenvironment can be decreased by treatment of that organ with agents that reduce oxidative stress and production of free radicals (Greenberger 1991; Demedts et al. 2005; Naparstek et al. 1985; Greenberger et al. 1988). The capacity of an irradiated tissue to favorably support the migration, homing, and stable functioning of bone marrow-derived cells, arriving from the circulation has been shown to be critically dependent on the functional status of the microenvironment of that target organ.
Irradiation damage to a specific organ includes both acute and chronic side effects (van Rongen et al. 1993). The eclipse or quiet phase between the disappearance of acute irradiation effects and appearance of late effects has been shown to be attributable to a second phase of cytokine production that is associated with the initiation of the late effects (Leask and Abraham 2004). It is generally accepted that for bone marrow-derived stem cells to have a functional role in tissue reconstitution of an epithelial organ, the deleterious effect of continuous free radical production by the microenvironment in that tissue must be ameliorated (Oberley and Buettner 1979). Research efforts have focused on development of techniques by which to supply stable antioxidant delivery to cells of the irradiated microenvironment to facilitate stem cell homing. At the present time, there still remain challenges for proof of the concept that bone marrow-derived cells can reconstitute an epithelial organ. These challenges include the demonstration that functional cellular physiologic units within the recipient’s irradiated tissue are derived from donor bone marrow cells. Some representative challenges include:
1.
Alveolar respiratory complexes in the lung must be shown to be of donor marrow cellular origin.
2.
Functioning muscle bundles producing muscle contraction and contributing to muscle strength must be shown to be associated with skeletal myocytes derived from donor bone marrow origin cells.
3.
Elements of glandular secretion/glandular buds and production of donor bone marrow genotype-derived secretary products must be shown to be derived from bone marrow genotype specific cells.
These criteria have been met in few situations including at present only the target recipient organs of liver and bone marrow itself (Wagers et al. 2002).
5 Bone Marrow-Derived Mesenchymal Stem Cells
Bone marrow fibroblasts were originally thought to be those cells supporting the homing and proliferation of hematopoietic cells (Friedenstein et al. 1982). Continuous bone marrow cultures provided a system by which to study cells of the hematopoietic microenvironment and their interaction with hematopoietic stem cells in vitro (Dexter et al. 1977). Hematopoietic stem cells derived from long-term bone marrow culture were shown to have the properties of freshly derived stem cells from donor mice (Dexter et al. 1977). Hematopoietic reconstitution of lethally irradiated mice by transplant of stem cells derived from long-term bone marrow cultures that had been in vitro for months, demonstrated the capacity of an in vitro culture system to contain persistent totipotential stem cells. Within the hematopoietic niche of these marrow cultures “cobblestone islands” were shown to contain quiescent cells that harbored true stem cells (Greenberger 1978; Mauch et al. 1980; Sakakeeny and Greenberger 1982). The aging of continuous bone marrow cultures was associated with decline in the number of stem cells and production of committed hematopoietic progenitors (Sakakeeny and Greenberger 1982). The long-term bone marrow culture system remains the only in vitro system for maintenance of true stem cells outside the body for long durations (Sakakeeny and Greenberger 1982).
Other organ explant systems have been shown to share some properties of the long-term bone marrow culture system (Kataoka et al. 2003; Kalabis et al. 2008; Engelhardt et al. 1993). The success of the long-term bone marrow culture system allowed focus on those cells of the microenvironment (nonhematopoietic) which were responsible for stable quiescence of hematopoietic stem cells (Mauch et al. 1980). Following explant to culture, long-term marrow cultures were shown to contain cells with endothelial markers, fibroblast markers, as well as macrophage markers (Sakakeeny and Greenberger 1982). The hematopoietic microenvironment was thought to be composed of these three cell types (Dexter et al. 1977). Accumulation of neutral fat/lipid in bone marrow stromal cell association of lipid containing droplets in stromal cells near hematopoietic niches, suggested that the adipocyte was the critical hemopoiesis supporting stromal cell (Greenberger 1978). Further information indicated that lipid accumulation was in fact the toxic response of bone marrow to the high concentrations of fat and corticosteroid in horse serum used to establish the cultures (Greenberger 1978). Cultures with limited fat accumulation led to longer duration of hematopoiesis (Sakakeeny and Greenberger 1982). This data was consistent with the clinical observation that accumulation of yellow fat occurs in bone marrow during aging, and the fatty repopulation of the marrow microenvironment was associated with hematopoietic failure (Sakakeeny and Greenberger 1982).
Ionizing irradiation effects on the hematopoietic microenvironment were shown to be similar to those in natural aging (Zhou et al. 2008). Irradiated cells accumulated fat more quickly (Greenberger 1991), irradiated cells in culture showed upregulation of p53 and p21, stress response elements also shown to be upregulated during aging of bone marrow stromal cells (Zhou et al. 2008). Factors which reversed fat accumulation and aging effects in bone marrow stromal cells were shown to enhance hematopoiesis (Lechpammer et al. 2005; Epperly et al. 2007).
There is evidence that the cells of bone marrow microenvironment can be repopulated/replenished by cells of donor bone marrow origin (Anklesaria et al. 1989; Werts et al. 1980). Replacement of the microenvironment was first demonstrated by Werts and Degowin (Werts et al. 1980) and confirmed by Anklesaria et al. (1989) who showed that bone marrow stromal cells engrafted less effectively to high dose irradiated sites compared to low dose irradiated sites. These cells were called bone marrow fibroblasts, but later were shown to be those cells capable of multilineage differentiation (Pittenger et al. 1999).
Bone marrow stromal cells can engraft into a fracture/bone wound healing defect in vivo (Gokhale et al. 2010) and they are involved in regeneration/osteogenesis in irradiation damage tissue. Higher irradiation doses limit the capacity of migratory cells to be involved in bone healing (Gokhale et al. 2010). Antioxidant administration facilitates improved mesenchymal stem cell engraftment. However, serious negative effect of bone marrow stromal cell engraftment is the role of these cells in late tissue irradiation damage. Bone marrow stromal cells have been shown to contribute significantly to the fibrosis in irradiated lung (Epperly et al. 2003). Bone marrow stromal cell involvement in fibrosis is also a factor in chemotherapeutic drug induced lung damage such as that by bleomycin injury (Ortiz et al. 2003). Under one set of conditions, bone marrow-derived cells can be involved in tissue repair and potentially organ reconstitution, and under another set of conditions a distinct mesenchymal stem cell subset can contribute to late fibrotic damage which limits organ recovery. The subtle dynamics of cell migration into an irradiated microenvironment can be either beneficial or deleterious depending on the cell population and the timing.
6 The Irradiated Tissue Microenvironment: Niche or Vascular Space?
It is clear that above a toxic threshold dose of irradiation (which may differ between tissues and organs) spontaneous tissue reconstitution may not follow. The protection or replacement by bone marrow cells of the irradiation damaged tissue microenvironment after such a toxic dose may lead to improved tissue recovery. There is controversy as to whether the microenvironment for stem cells is in fact a vascular space (Lombaert et al. 2008) or a specific cell type which functions as the niche (Anklesaria et al. 1989; Adams et al. 2007; Zhang et al. 2003). There is further controversy over whether the cells of the microenvironment as well as the stem cell population itself can be replaced by bone marrow.
Reconstitution of irradiated epithelial and nonepithelial tissues is dependent upon a subtle interplay between migratory beneficial cells (tissue reparative stem cells) and deleterious late damage inducing cells (fibrosis inducing mesenchymal stem cells). One approach toward tipping the balance toward repair may be to limit oxidative stress responses in the irradiated tissue microenvironment which change the makeup of cytokine production and release. Therefore, in addition to marrow-derived stem cell transplant, local delivery of antioxidants by stimulation of the in situ replenishment of antioxidant stores, by specific antioxidant diets, or by direct perfusion with antioxidants may facilitate tissue repair. Research is ongoing and important to achieve the goal of replacing irradiation-damaged tissue through donor stem cell transplantation.
References
Aird WC, Jahroudi N, Weiler-Guettler H, Rayburn HB, Rosenberg RD (1995) Human von Willebrand factor gene sequences target expression to a subpopulation of endothelial cells in transgenic mice. Proc Natl Acad Sci U S A 92:4567
Albera C, Polak JM, Janes S, Griffiths MJD, Alison MR, Wright NA, Navaratnarasah S, Poulsom R, Jeffrey R, Fisher C, Burke M, Bishop AE (2005) Repopulation of human pulmonary epithelium by bone marrow cells: a potential means to promote repair. Tissue Eng 11:7–8CrossRef
Anklesaria P, FitzGerald TJ, Kase K et al (1989) Improved hematopoiesis in anemic S1/S1d mice by therapeutic transplantation of a hematopoietic microenvironment. Blood 74:1144–1152PubMed
Ara T, Tokoyoda K, Sugiyama T, Egawa T, Kawabata K, Nagasawa T (2003) Long-term hematopoietic stem cells require stromal cell-derived factor-1 for colonizing bone marrow during ontogeny. Immunity 19:257–267PubMed
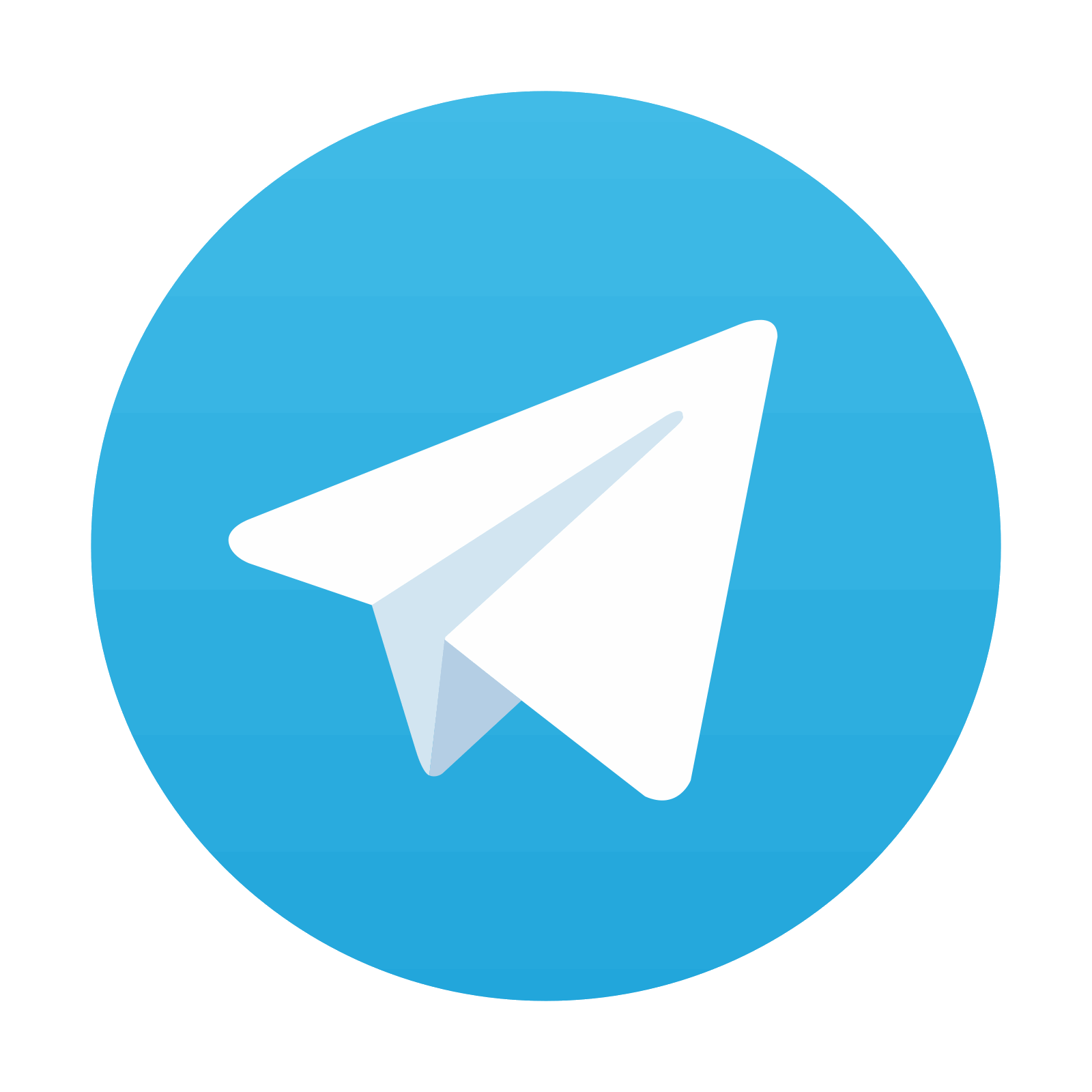
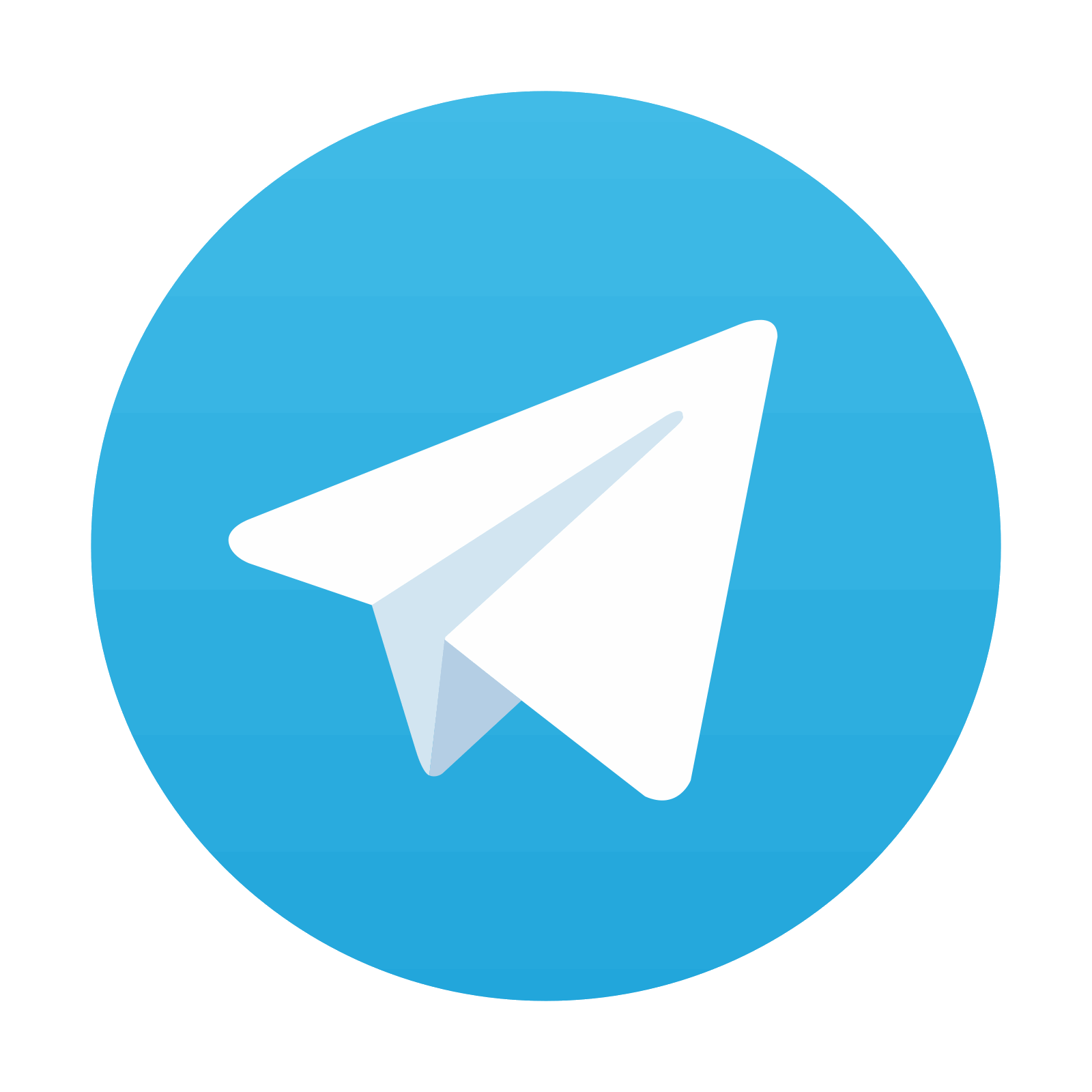
Stay updated, free articles. Join our Telegram channel
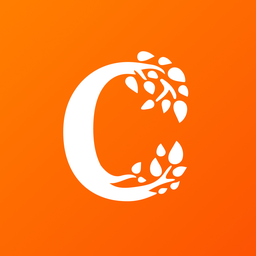
Full access? Get Clinical Tree
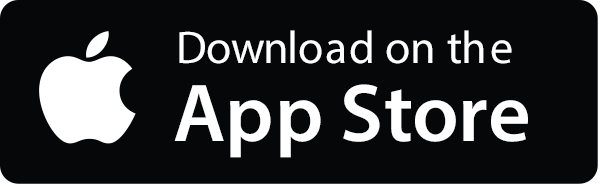
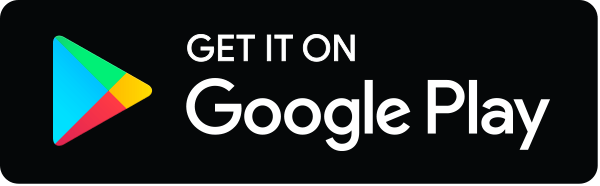
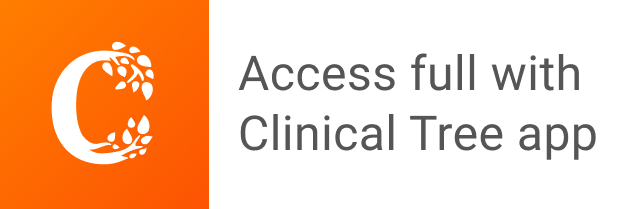