Shape and architecture
Subcategory
Example
Fibres parallel to the line of pull
Strap-like
Sartorius
Tendinous intersections at intervals
Rectus abdominis
Fusiform muscle—close to parallel in the belly. Converge on a tendon either proximally, distally or both
Biceps femoris
Fibres oblique to the line of pull
Triangular
Adductor longus
Unipennate
Flexor pollicis longus
Bipennate
Rectus femoris
Multipennate
Deltoid
Spiral or twisted arrangement
Sternal fibres of pectoralis major, latissimus dorsi
Passes obliquely from superficial to deep aponeuroses (“unipennate” type)
Soleus
Spiral around a bone
Supinator
Two or more planes of fibres arranged in differing directions
Sternocleidomastoid
When a muscle fibre is viewed microscopically, transversely orientated bands or striations are identified due to differences in refractive indexes of the various parts of the sarcomere. Z-lines constitute the boundaries of the sarcomere. The A-band consists of thick filaments with interdigitation and a degree of overlap of the thin filaments at each end. The I-band consists of two adjacent sarcomeres where the thin filaments do not overlap the thick filaments (Standring 2005).
Nuclei of the muscle fibre are located at the periphery, under the plasma membrane or sarcolemma and are most numerous in the region of the neuromuscular junction. Also located adjacent to the nuclei and to a lesser extent, adjacent to the myofibrils, are Golgi apparatus, ribosomes and mitochondria.
The endomysium is a delicate network of connective tissue, which surrounds muscle fibres. It contains capillaries and bundles of small nerve fibres and is the site of metabolic exchange between muscle and blood.
The perimysium covers groups of muscle fibres to form parallel bundles called fasciculi. The perimysium is the inward extension of the epimysium, which is a collagenous sheath that invests entire muscle groups. All three sheaths (endomysium, perimysium and epimysium) coalesce at sites of attachment to tendons, aponeuroses and fasciae, thereby creating the ability to resist shear stresses (Standring 2005).
Muscle fibres are functionally divided into two groups—type I (also known as slow-twitch fibres) and type II (also known as fast-twitch fibres). Type II fibres are further subdivided into types IIa, IIx and IIc. The human body consists of 50 % type I, 25 % type IIa, 23–24 % type IIx and 1–2 % type IIc fibres (Exeter and Connell 2010).
Type I fibres have a longer time to reach peak tension (110 ms), compared with type II fibres (50 ms). Type II fibres are larger, contain a faster form of ATPase and have a more developed sarcoplasmic reticulum, ensuring more effective calcium delivery. Different fibre types also contain a variety of different ATP generation mechanisms for muscle contraction—type I fibres are slow oxidative, type IIa are fast oxidative/glycolytic, type IIx are fast glycolytic (Exeter and Connell 2010).
Neural innervation also differs between fibre types. The type of motor neuron that innervates a specific group of muscle fibres determines the muscle fibre type. Each motor unit (varying number of muscle fibres) innervates only one fibre type (Standring 2005).
Specific muscles and muscle groups throughout the body are therefore able to perform certain functions depending on the type of muscle fibres they contain. Type I fibres are activated during low intensity exercise or endurance events and produce less force than type II fibres. Type II fibres are recruited during short duration, high intensity activities. Aerobic, anaerobic or resistance training is capable of changing fibre types (Standring 2005).
1.2 Tendons
Tendons facilitate the transmission of forces generated by muscle contraction on to bone, facilitating movement and maintenance of posture. Tendons are present at the insertion of most muscles. Many muscles also have a tendinous origin. Tendons are round or oval in cross-section and consist of fascicles of type I collagen, orientated parallel to the long axis of the tendon. Small vessels and nerves course within loose connective tissue between fascicles.
Tendons attach to bone, both at the periosteum and through fasciculi that continue deep into the cortex of the bone. A plate of fibrocartilage at the site of bony attachment serves to cushion and reinforce the site of attachment (Standring 2005). Tendons vary in length in different muscles and may have an intramuscular component or component at the muscle surface, resulting in a relatively elongated muscle tendon junction.
The elasticity of tendons allows them to be elongated by up to 6 % without damage. Recovery of the elastic recoil of tendons may also make movement more efficient (Standring 2005).
1.3 Aponeuroses
Aponeuroses are flattened tendons (Benjamin et al. 2008) which may be separate structures emerging from a muscle belly (e.g. tendons of latissimus dorsi and pectoralis major) or fibrous sheet on the surface or within a muscle (e.g. soleus). Strain within the tendon of soleus differs in the aponeurotic portion when compared with the remainder of the tendon (Finni et al. 2003). This is associated with the pattern of force transmission via intramuscular connective tissue. Non-homogeneous strains may occur within the aponeurosis of soleus, which may be secondary to a compartmentalised recruitment of muscle fibres for a submaximal contraction (Finni et al. 2003).
1.4 Muscle–Tendon junction
The muscle–tendon junction (musculotendinous or myotendinous junction) is the weakest link in the muscle tendon unit (Palmer et al. 1999). At the muscle–tendon junction, tendon fibrils insert into deep recesses between finger-like processes of skeletal muscle cells, which are covered by a thick layer of basement membrane (Jozsa and Kannus 1997). The tendon fibrils attach to the basement membrane of the skeletal muscle cells.
Interdigitation between the tendon fibrils and myocytes increases the contact area between myocytes and extracellular collagen fibres and results in the ability to dissipate enormous tensile forces during muscle contraction (Palmer et al. 1999). Unfortunately, when compared with the adjacent muscle, tendon and osseous insertion, the muscle–tendon junction has poorer viscoelastic properties and a reduced capacity for energy absorption (Palmer et al. 1999). As a consequence, in adults, the most common site of muscle strain or tear is at the muscle–tendon junction.
2 Patterns of Traumatic Injury
2.1 Introduction
Muscle injuries are one of the most common injuries sustained while playing sport and constitute between 10 and 55 % of all sporting injuries (Beiner and Jokl 2001; Best and Hunter 2000; Garrett 1996; Huard et al. 2002; Järvinen et al. 2005). Muscle injury results from a variety of mechanisms including muscle strain/tear, epimyseal fascial injury, delayed onset muscle soreness (DOMS), muscle contusion, muscle herniation and lacerations (Kijowski 2011; Kneeland 1997). Avulsions of the origin or insertion of muscles and tendinopathy is beyond the scope of this chapter.
Mimics of muscle injury on magnetic resonance imaging include—subacute denervation, muscle infarction, inflammatory myositis, normal immediate post exercise muscle oedema and deep venous thrombosis (Farber and Buckwalter 2002).
2.2 Acute Tensile Overload
Muscle strain is the most common muscle injury sustained by both the general and sporting populations (Blankenbaker and Tuite 2010; Linklater et al. 2010; Shelly et al. 2009; Slavotinek 2010). Muscle strains occur when the muscle is subjected to an excessive tensile force, which leads to overstretching and rupture of myofbrils (Kijowski 2011). Most commonly, muscle strains occur during eccentric contraction. During an eccentric muscle contraction, muscle fibres are forced to lengthen, creating greater tensile forces than can be created during concentric contraction, when muscle fibres shorten. Injuries predominantly occur at the musculotendinous junction and may involve the intramuscular tendon.
The majority of muscle injuries are diagnosed clinically (Kijowski 2011). There is a spectrum in the clinical presentation of muscle strain injury, which may range from a severe tearing sensation to a mild tightening sensation. Historically, the most commonly used grading system was devised by O’Donoghue (1962) on the basis of the clinical impression of the extent of injury. Grade 1 injury (strain) consists of a minor degree of microscopic tearing with no macroscopic muscle fibre discontinuity. Grade 2 injury (partial tear) is defined as an incomplete disruption of muscle fibres. Clinically, grade 1 and grade 2 injuries can be difficult to distinguish—both grades result in loss of function and in some studies have shown no significant difference in time to return to sport (Blankenbaker and Tuite 2010). Grade 3 injury (complete tear) is a complete rupture of the muscle. This grading system fails to take into account the presence or absence of concomitant tendon injury and if present, the extent of tendon injury. There is scope for development of a new classification system which more precisely characterises the extent of muscle and tendon injury, hopefully providing a higher level of prognostic significance with respect to time to return to sport and risk of recurrent tear (Linklater et al. 2010).
There are specific characteristics of a muscle that makes it more susceptible to injury. Long, fusiform muscles that extend across two joints and have a propensity for eccentric contraction are more susceptible to injury. Examples of these muscles include biceps brachii, rectus femoris, long head of biceps femoris, semitendinosus and semimembranosus (hamstrings) and the medial head of gastrocnemius. A predominance of type II fibres may also predispose to injury.
Other factors which may predispose to muscle tears include: past muscle strain injury, low muscle strength, muscle fatigue, age, lack of warm-up, muscle temperature and poor flexibility (Garrett 1996; Mair et al. 1996; Orchard 2001).
The long head of biceps femoris, semimembranosus, and semitendinosus muscles consist of a proximal and distal “free” tendon and relatively extensive tendon–muscle interface which may be intramuscular (central tendon) or at the muscle surface, such that the proximal and distal tendons overlap or almost overlap. The central tendon is similar in configuration to the central rachis of a feather, with radiating myofibrils arising from it, exemplified by the proximal tendon of long head biceps femoris (Comin et al. 2013). As stated previously, the most common site of muscle strain/tear occurs adjacent to the muscle–tendon junction, which may be located at the central aspect of the muscle or eccentrically at the muscle surface (Blankenbaker and Tuite 2010; Noonan and Garrett 1992) (Fig. 1). A study by Weishaupt (2001) demonstrated that 33 % of hamstring tears occur at the proximal muscle–tendon junction, 53 % at the intramuscular muscle–tendon junction and 13 % at the distal muscle–tendon junction (Weishaupt et al. 2001). These findings therefore indicate that the most common site of muscle–tendon junction injury is within its intramuscular portion.
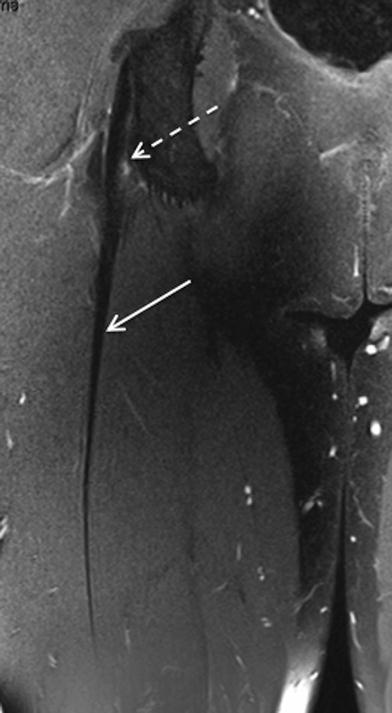
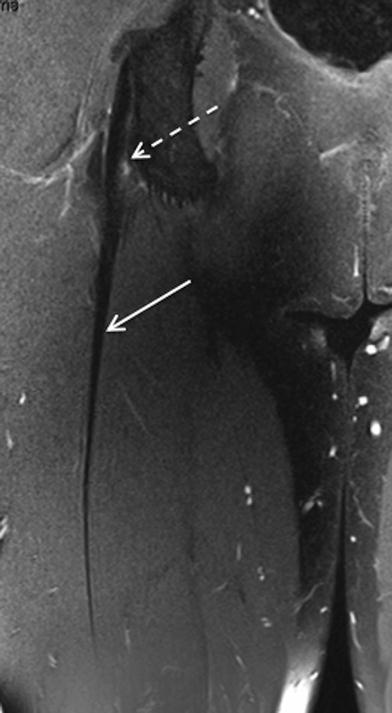
Fig. 1
Coronal proton density weighted MR image with fat saturation demonstrates the normal intramuscular component of the proximal tendon of the long head biceps femoris (long arrow). Note the presence of hamstring origin tendinopathy and non-acute, non-retracted deep surface partial thickness tear (short arrow)
In a study by (Comin et al. 2013), central tendon disruption occurred exclusively in biceps femoris injury (12 of 45 cases). There were no central tendon disruptions of the semimembranosus or semitendinosus. In this same study, recovery times for injuries to the biceps femoris were significantly greater if there was central tendon disruption (Comin et al. 2013).
The investing fascia of a muscle can be described as a fascial ectoskeleton, providing an additional anchor point for muscle fascicles in addition to the proximal and distal tendons. Peripheral/epimyseal muscle injuries adjacent to the investing fascia/epimysium are less common than muscle–tendon junction injuries (Kijowski 2011). When they occur, they are thought to relate to differences in elasticity between epimysium and the adjacent muscle fibres (Blankenbaker and Tuite 2010). This is similar in pathogenesis to injuries at the muscle–tendon junction (Koulouris and Connell 2005). Peripheral/epimyseal injury has been described in the quadriceps and hamstring muscles, caused by an eccentric contraction (Connell et al. 2004; Cross et al. 2004). Differential contraction in two adjacent muscles may lead to injury involving both muscles.
2.3 Muscle Contusion
Muscle contusions constitute 2 % of muscle injuries, most commonly involving the quadriceps muscle group. They occur when a muscle sustains a sudden blunt force/direct blow, causing compression of the deep portion of the muscle against the underlying bone (Järvinen et al. 2005). As a result, there is disruption of capillaries and muscle fibres and an intramuscular haematoma which forms between muscle fibres, without muscle fibre disruption in most cases. Occasionally direct blunt trauma may result in muscle fibre discontinuity, for example in surfboard injuries to the biceps brachii muscle.
Contusional injuries are typically deep within the muscle belly, often involve more than one muscle and tend to be less symptomatic and have a shorter clinical course compared to muscle strain injuries. Clinically there are three severity grades described: mild, moderate and severe, depending on the remaining range of motion (Jackson and Feagin 1973). The loss of range of motion does not necessarily correlate with the extent of the haematoma on imaging and does not always correlate with loss of function (Megliola et al. 2006).
2.4 Delayed Onset Muscle Soreness
DOMS is an indirect muscle injury with reversible structural damage to the muscle at a cellular level resulting in an acute inflammatory response and an increase in intracellular fluid (Armstrong 1984; Cleak and Eston 1992). DOMS is associated with eccentric muscle contraction and is directly related to the intensity and duration of physical activity (Cleak and Eston 1992). Acute grade 1 muscle strain may be differentiated from DOMS clinically. Grade 1 strains have an acute onset of pain and dysfunction. Symptoms associated with DOMS typically commence at 1–2 days after the causative activity, is greatest at 24–48 h and gradually resolves over the next 48–72 h (Armstrong 1984; Cleak and Eston 1992).
2.5 Pressure Overload (Compartment Syndrome)
A compartment syndrome is elevation of pressure within a confined anatomic space resulting in a reduction in blood flow that threatens the viability and function of the tissues within the compartment (Stedman 2000). Compartment syndromes may present as a complication of an acute muscle injury. The most common causes include: fracture, blunt or penetrating trauma, burns, intramuscular haemorrhage and less likely, secondary to muscle tear. Patients commonly present with pain, which is disproportionate to the injury, exacerbation of pain when the affected muscle group is passively stretched. Neurological symptoms may also be present (Arciero et al. 1984; Davies 1979; Pearl 1981). Acute compartment syndrome is a surgical emergency requiring exploration and fasciotomy. In contrast, chronic exertional compartment syndrome involves exercise related pain which resolves with rest.
2.6 Muscle Hernia
Muscle hernias are an uncommon complication of muscle/compartmental injury and represents herniation of muscle through a small fascial defect which may relate to prior penetrating injury or develop at a site of penetration of the investing fascia by a nerve (e.g. superficial peroneal nerve as it penetrates the investing fascia of the lateral compartment in the leg).
3 Muscle Healing
Skeletal muscle healing occurs in a predictable sequence, regardless of the underlying cause of the injury (Hurme et al. 1991; Kalimo et al. 1997). The ruptured myofibres contract and the gap fills with blood products. An inflammatory response develops, with neutrophils and macrophages infiltrating the site of injury.
Release of proteases results in localised myofibre breakdown and necrosis. Macrophages then remove cell debris and secrete growth factors. The basement membrane remains intact, which subsequently acts as a scaffold that facilitates anatomically aligned reconstitution of the myofibrils.
Growth factors activate the regenerative stem cells of the muscle (called satellite or progenitor cells) which form myoblasts. Myoblasts differentiate into mononucleate myoblasts, then fuse to form multinucleated myotubes and finally undergo maturation to become myofibres (Hurme et al. 1991).
The ends of the repaired myofibres do not reattach to each other. Each ruptured myofibre attaches to, and is separated by interposed scar tissue produced by myofibroblasts (Hurme et al. 1991; Kalimo et al. 1997). A remodelling phase occurs, which is a period of maturation of the regenerated myofibres, retraction and reorganisation of the scar tissue and revascularisation with ingrowth of capillaries into the reparative tissue (Järvinen et al. 2005).
When there is a significant sized muscle tear, in the long term, there may be focal muscle fatty atrophy at the margins of the scar tissue and an overall reduction in muscle volume (Kääriäinen et al. 1998; Silder et al. 2008).
The single most important determinant of subsequent muscle function and risk of reinjury is the quality of scar formation and remodelling (Clanton and Coupe 1998; Stauber 1989). Scar formation may inhibit innervation of regenerating muscle tissue and reduce muscle contractility and range of motion (Quintero et al. 2009). The developing scar is the weakest point for recurrent tears up to 12 days post injury. After this time, re-tears occur in the adjacent myofibrils at the margins of the scar (Järvinen et al. 2000).
4 Complications of Muscle Injury
4.1 Introduction
Complications of acute muscle injury include—haematoma, acute compartment syndrome, myositis ossificans and calcific myonecrosis (Counsel and Breidahl 2010).
4.2 Myositis Ossificans/Heterotopic Ossification
Myositis ossificans is extensively presented in “MRI of muscle tumours” and thus here only briefly discussed in the direct context of muscle injuries.
As a complication of muscle injuries, heterotopic ossification occurs as myositis ossificans circumscripta. Following a muscle tear or a direct contusion and sometimes without a remembered trauma, a haematoma is initially present. This haematoma typically undergoes a maturation process exhibiting characteristic clinical, radiological and histological features.
4.3 Calcific Myonecrosis
Calcific myonecrosis presents as an expanding calcific mass that develops within a muscle post trauma. Most reported cases have occurred in the anterior and lateral compartments of the lower leg, with only single cases reported in the foot and forearm (Holobinko et al. 2003; Larson et al. 2004).
This entity most commonly occurs due to compartment syndrome post fracture of the tibia or femur and more rarely after neurovascular injury (Early et al. 1994; Milisano and Hunter 1992; Renwick et al. 1994; Viau et al. 1993). The reported interval from injury to presentation ranges from 10 to 64 years (average, 36 years) (Wang and Chen 2001).
The pathogenesis of calcific myonecrosis is unknown. An entire single muscle is replaced with central liquefaction and peripheral calcification.
The radiographic findings demonstrate a fusiform mass, which involves the entire muscle, with linear, peripherally oriented, plaque-like amorphous calcification. Differential diagnoses on radiographs include tumoral calcinosis, calcific tendonitis, calcific bursitis, synovial osteochondromatosis, synovial sarcoma, chondrosarcoma, osteosarcoma, and myositis ossificans (Olsen and Chew 2006).
5 Imaging Modalities and Technique
5.1 Introduction
The role of imaging in acute muscle injuries includes establishing the site and extent of injury and providing some prognostic guidance regarding return to sport. In general the threshold for imaging is substantially lower in the elite athlete, when compared to the recreational athlete. In the recreational athlete, depending on the clinical context, there may still be a role for imaging to exclude an injury requiring surgery such as a hamstring origin avulsion (Colosimo et al. 2005; Cross et al. 2004; Folsom and Larson 2008; Slavotinek et al. 2002). In the elite athlete there may be a role for imaging in monitoring healing of a muscle injury.
Both MRI and ultrasound (US) may be utilised for the diagnosis of muscle injury (please see also “Imaging the skeletal muscle – when to use MRI and when to use ultrasound”). In Europe, US is often used as the first-line imaging modality. Ekstrand et al. (2012) have investigated which imaging methods are used to investigate hamstring injuries in 23 European professional soccer teams and revealed the following distribution: 58 % were examined by MRI, 29 % by US only and 40 % by both MRI and US. Interestingly, 13 % were managed clinically without the use of imaging. In North America and Australia, MRI is more commonly utilised in the first line assessment of acute muscle injury. The accuracy of MRI is superior to US, particularly with deep muscle injuries (Guillodo et al. 2011).
5.2 Magnetic Resonance Imaging
5.2.1 Introduction and Pulse Sequences
MRI is the “gold standard” for investigation of muscle injuries (Hayashi et al. 2012). Fluid sensitive sequences (Short Tau Inversion recovery (STIR), Dixon or frequency selective fat suppressed Proton Density (PD) or T2 sequences) are most efficacious in rendering conspicuous the intramuscular oedema associated with acute muscle injury, in demonstrating muscle fibre discontinuity and tendon injury. Fluid sensitive sequences are often combined with either a non-fat suppressed PD or T1 sequence. Measures of the longitudinal lengths as well as percentage and volume of muscle injury (Connell et al. 2004) in MRI are strong predictors of the time to return to play. It has been suggested that persistence of oedema defines the period of increased vulnerability to develop a re-tear (Fleckenstein et al. 1989). MRI is also more sensitive in monitoring muscle healing compared with US (Fleckenstein et al. 1989).
As a consequence, MRI may be of benefit for the assessment of the nature and extent of the injury, particularly in elite athletes.
The normal MRI appearance of muscle is intermediate signal intensity on all sequences. There should be no signal hyperintensity on water sensitive fat-suppressed, inversion recovery or Dixon subtraction method techniques (Linklater et al. 2010) (Fig. 2).
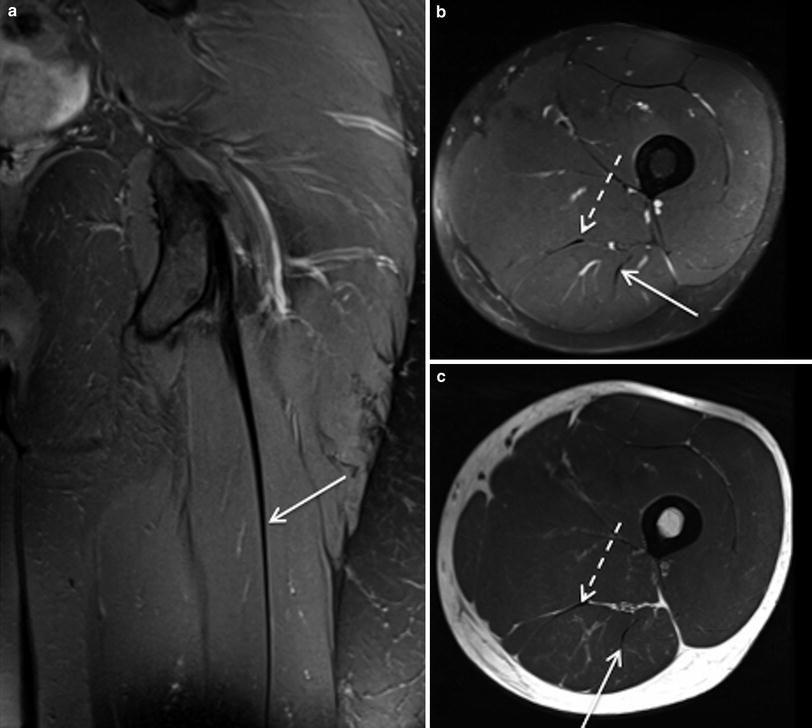
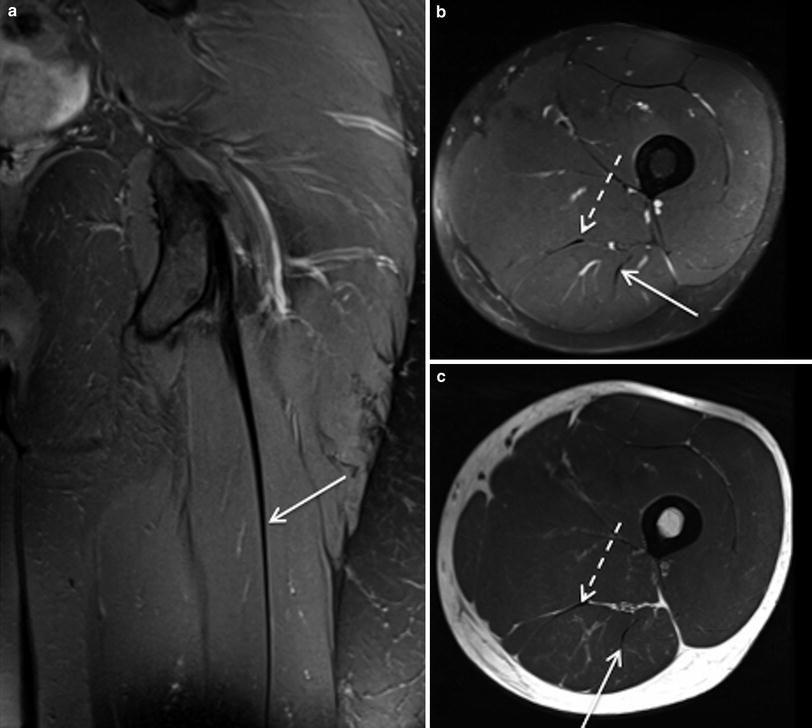
Fig. 2
Coronal proton density weighted MR image with fat saturation (a) and axial proton density weighted MR images, with and without fat saturation (b and c respectively) demonstrates the normal proximal tendon of long head biceps femoris (arrows) and semimembranosus (dashed arrows)
Prior to imaging, a skin maker should be placed on the patient overlying the region of interest. The coverage of the study should include the muscle origin, extending below the region of abnormality. Selection of an appropriate coil should be based on the coverage required, so that the coil does not need to be repositioned during the study. Pulse sequences should include: long and short axis proton density (PD) and either a STIR or frequency selective fat suppression (T2 or proton density) or Dixon fat suppression technique. A single long axis T1 sequence is also performed in some centres for characterisation of haematomas and identification of muscle atrophy.
Muscle fibre tracking with diffusion tensor imaging (DTI) has been used to assess muscle damage (Zaraiskaya et al. 2006) and to measure pennation angle (Lansdown et al. 2007). Normal muscle on DTI demonstrates uniformity in bulk directionality and exhibits orderly arrangements (Hayashi et al. 2012). Disturbance of normal arrangement of muscle fibres is demonstrated on DTI after injury (O’Brien 2005). At present, DTI remains an investigation/research tool and does not play a role in the clinical MRI assessment of muscle injury (see also “Diffusion-weighted and diffusion-tensor imaging: Applications in skeletal muscles”).
5.2.2 MRI Findings of Acute Muscle Strain/Tear
Grade I muscle strain demonstrates intramuscular signal hyperintensity on fluid sensitive sequences without discernible muscle fibre disruption. The oedema pattern is classically “feathery” in appearance (Blankenbaker and Tuite 2010). This represents oedema insinuating between intact muscle fascicles. The injury is most commonly seen adjacent to the muscle–tendon junction but may be peripheral-epimyseal. Fluid may also be identified tracking along the perifascial intermuscular region. In professional sport, the threshold for performing an MRI for muscle strain injury is reducing. As a consequence, there are increasing instances where it is difficult to differentiate between post exercise oedema and a grade 1 strain (Figs. 3, 4). In general post exercise oedema demonstrates a ground glass-like, mild degree of signal hyperintensity and often demonstrates a somewhat patchy geographic distribution (Fig. 5).
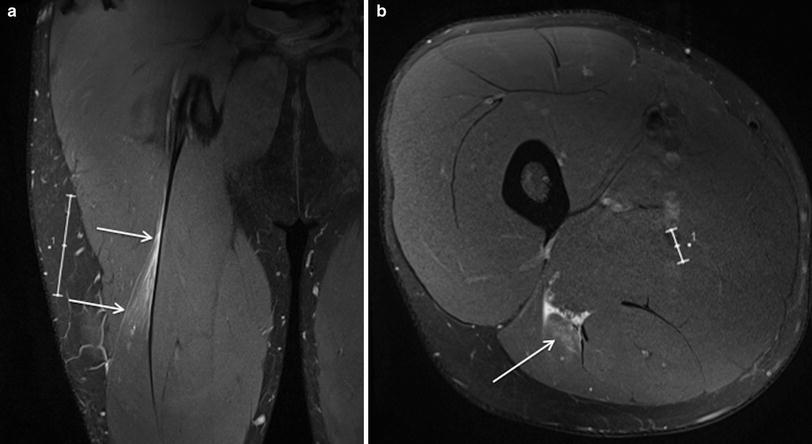
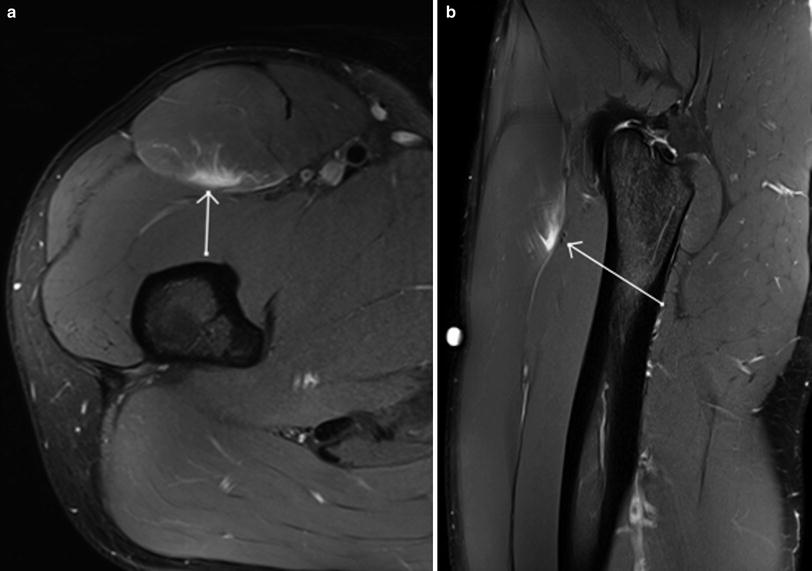
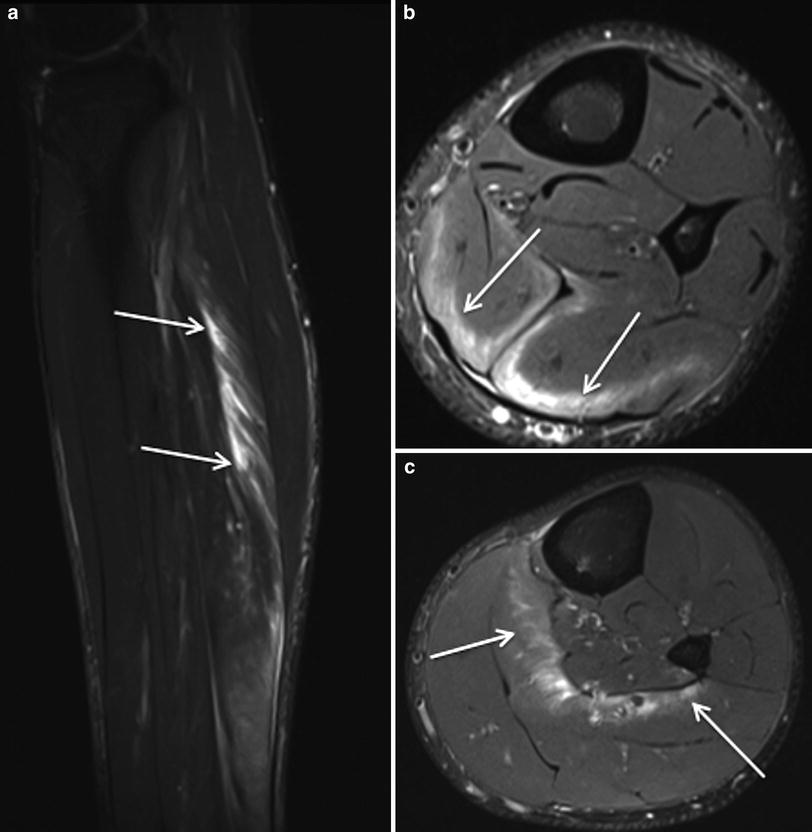
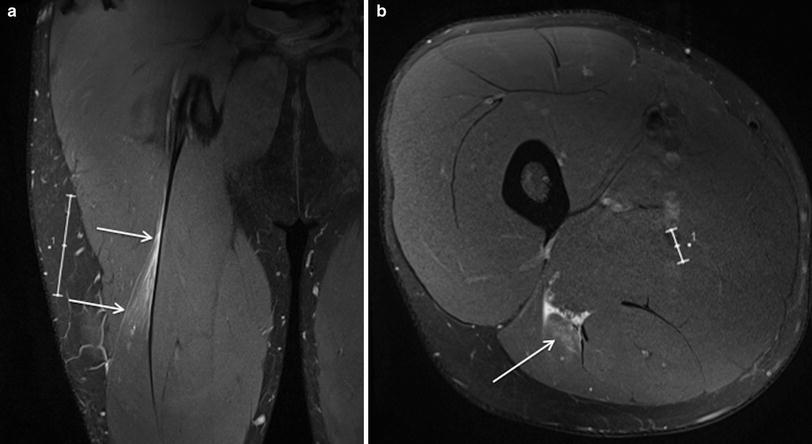
Fig. 3
Coronal (a) and axial (b) proton density weighted MR images with fat saturation in an Australian Rules football player with grade 1 muscle strain injury centred on the proximal muscle-tendon junction of the long head biceps of femoris, manifest as mild feathery intramuscular oedema and subfascial and perifascial oedema, without muscle fibre discontinuity or acute tendon injury (arrows). Note the presence of mild scarring of the intramuscular segment of the tendon related to remote injury
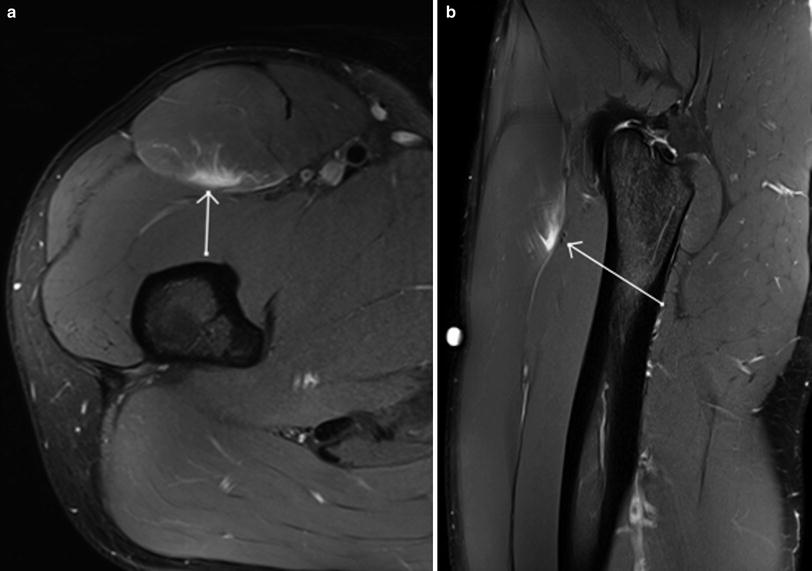
Fig. 4
Axial (a) and sagittal (b) proton density weighted MR images with fat saturation show a grade 1 epimyseal muscle strain injury of rectus femoris, manifest as eccentric-peripheral muscle oedema, without muscle fibre discontinuity (arrows)
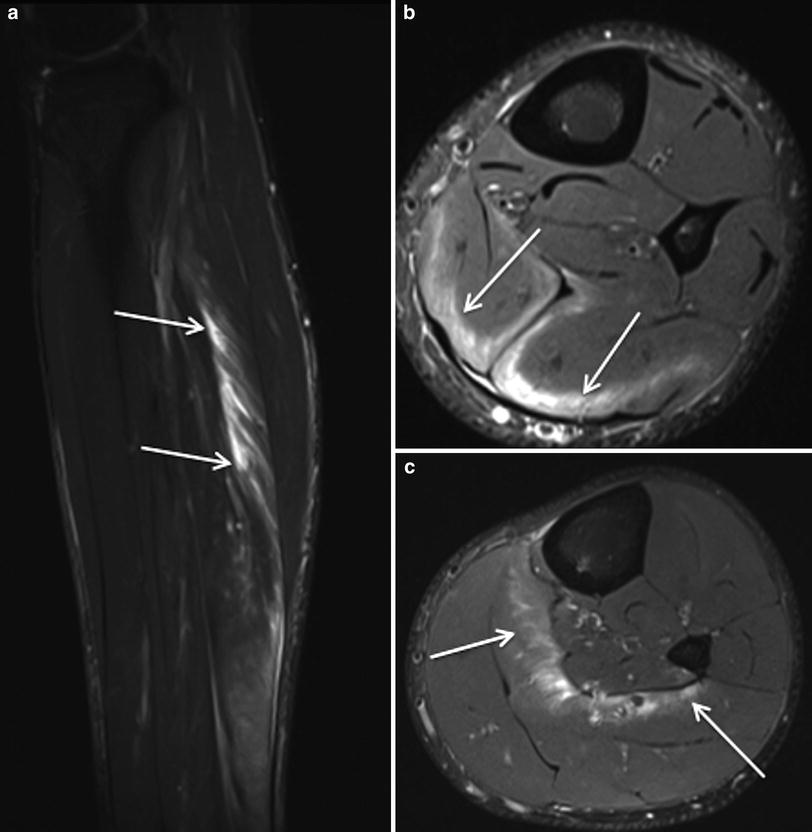
Fig. 5
Sagittal STIR MR image (a) and axial proton density weighted MR images with fat saturation (b and c) demonstrate extensive post exercise oedema within the soleus muscle in an Australian Rules football player, centred around the muscle tendon junctions, demonstrating a somewhat geographically marginated distribution (arrows). Note: The patient played an entire game 2 days after this MRI
In partial tears (grade 2), disruption of muscle fibres is identified. Oedema and haemorrhage is present within the muscle or at the muscle–tendon junction, often with perifascial extension (Kijowski 2011). Concomitant injury to the intramuscular component of the tendon may be evident, manifest as signal hyperintensity on fluid sensitive sequences. On longitudinal cross-section the tendon may demonstrate nonlinear, concertina-like morphology due to elongation. Higher grade injuries may be associated with macroscopic tendon fibre discontinuity (Kijowski 2011). A haematoma at the muscle–tendon junction may develop in patients with a partial tear and is generally not seen in the setting of a Grade 1 strain injury (El-Khoury et al. 1996). Intramuscular haematomas are not always indicative of a Grade 2 muscle strain injury as they may also be seen in the setting of a contusional muscle injury (Figs. 6, 7, 8).
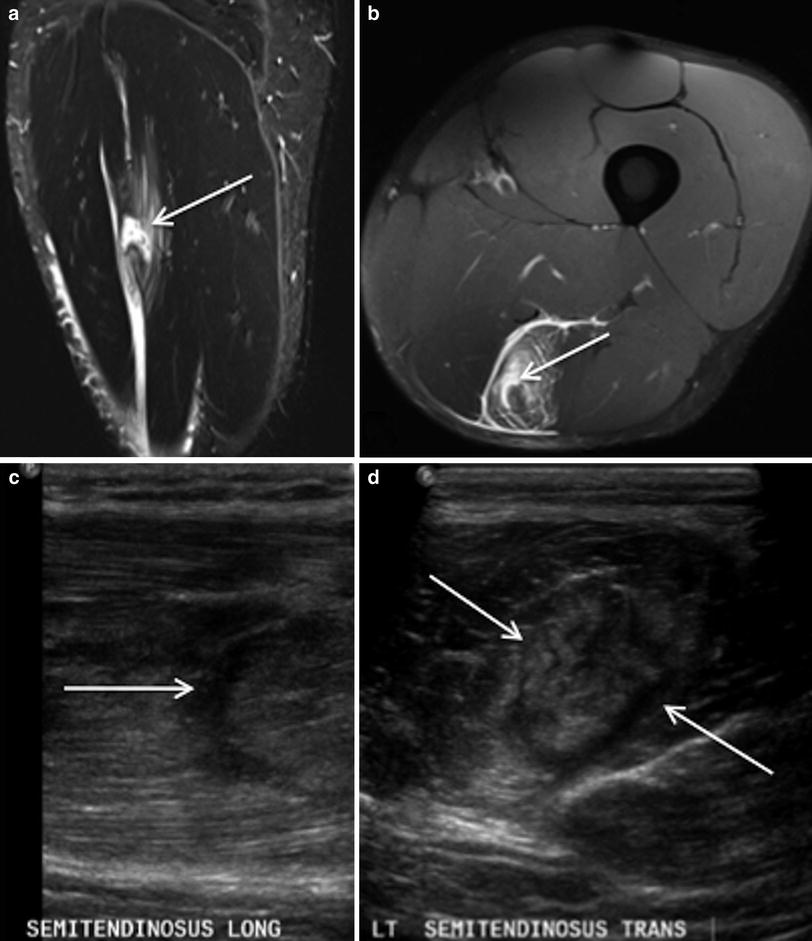
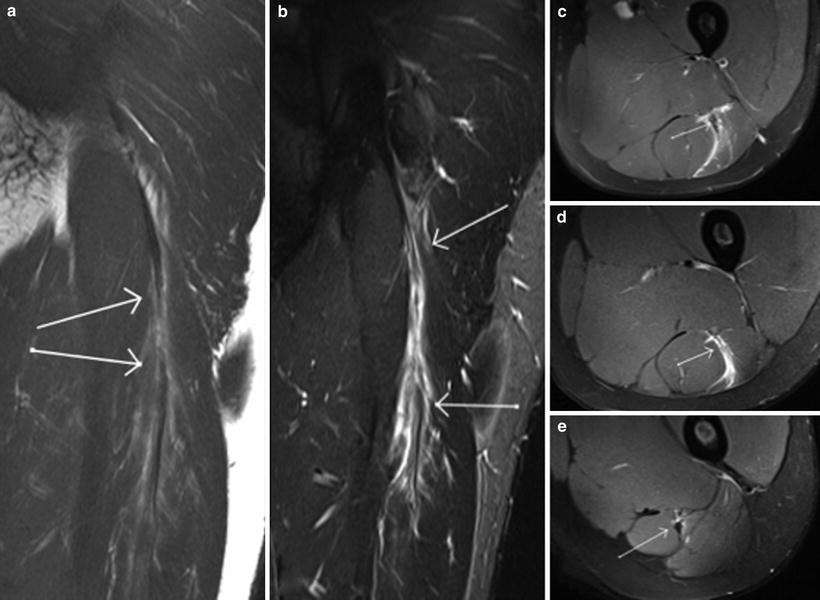
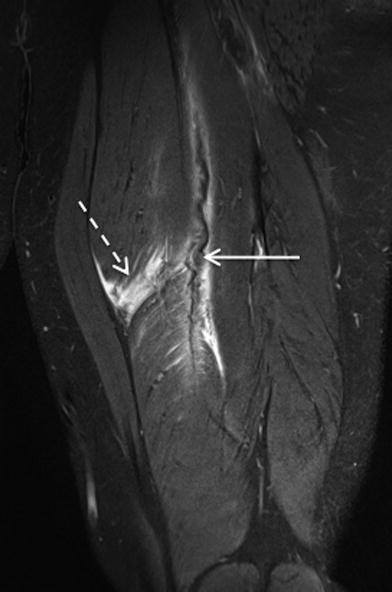
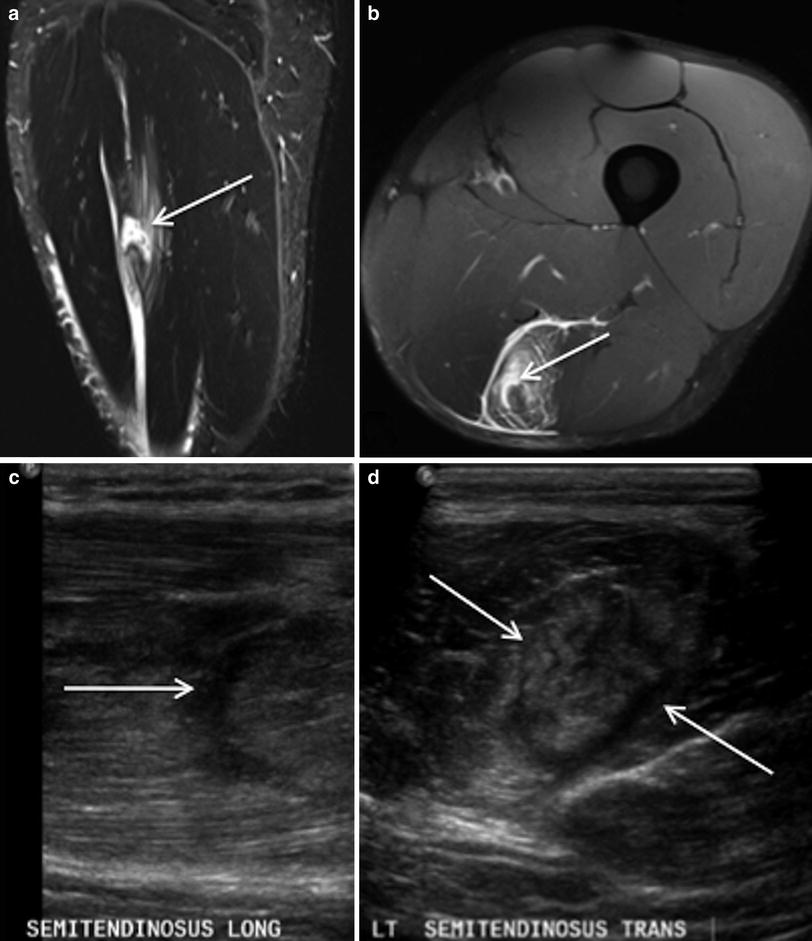
Fig. 6
Sagittal STIR MR image (a), axial proton density weighted MR image with fat saturation (b) and corresponding US images (c and d) in a professional rugby league player demonstrates a grade 2 injury of the semitendinosus muscle at the proximal margin of the intramuscular segment of the distal tendon, with fluid signal evident at the point of muscle fibre disruption and moderate surrounding intramuscular oedema (arrows). The area of muscle fibre discontinuity can also be appreciated on US. There was no associated tendon
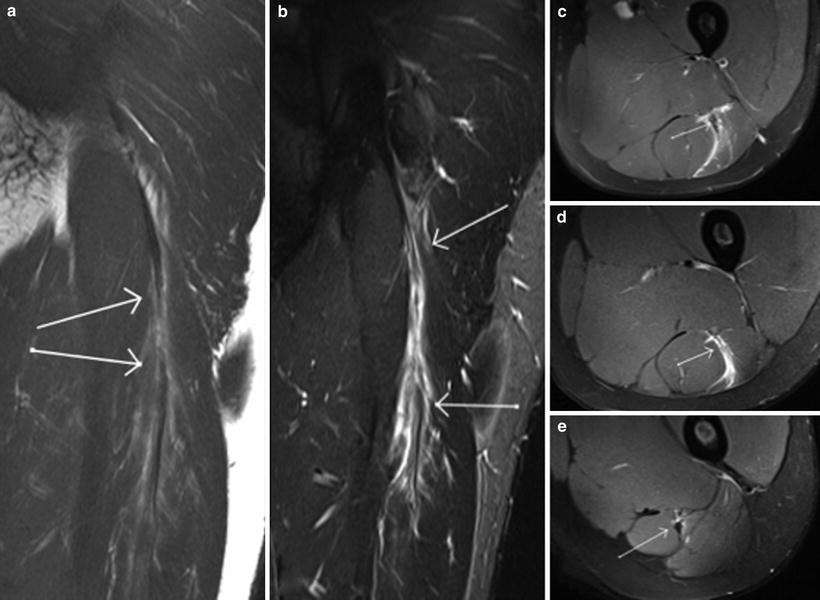
Fig. 7
Coronal STIR MR image (a), coronal T2 weighted MR image with fat saturation (b) and axial proton density weighted MR images with fat saturation (c–e) demonstrate an injury to the proximal aspect of the long head biceps femoris with frank tear of the intramuscular segment of the proximal tendon and adjacent feathery intramuscular oedema (arrows). This pattern of injury does not readily fit into the generally used classification system for muscle injuries which does not take into account the presence or absence of tendon injury
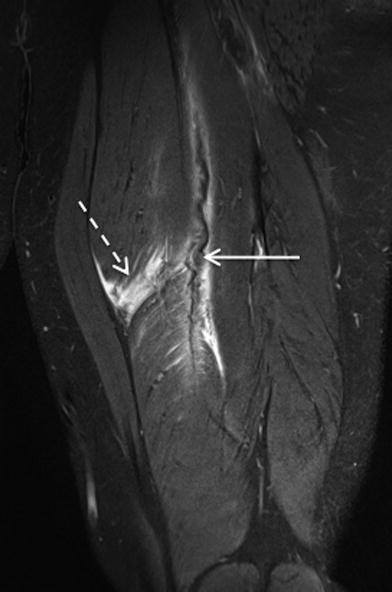
Fig. 8
Coronal proton density weighted MR image with fat saturation demonstrates a non retracted tear (arrow) of the intramuscular segment of the proximal tendon of the semimembranosus muscle, with mildly redundant morphology of the tendon at the proximal and to a lesser extent at the distal margin, with moderate adjacent intramuscular oedema and small focus of muscle fibre discontinuity (dashed arrow)
Complete tears (grade 3) demonstrate complete discontinuity of muscle fibres, usually with associated tendon fibre discontinuity and an associated haematoma. Extensive adjacent intramuscular and perifascial oedema may be present. It may be difficult to determine whether there is a partial or a complete tear due to blood and oedema filling the defect between the torn muscles (Blankenbaker and Tuite 2010) (Fig. 9).
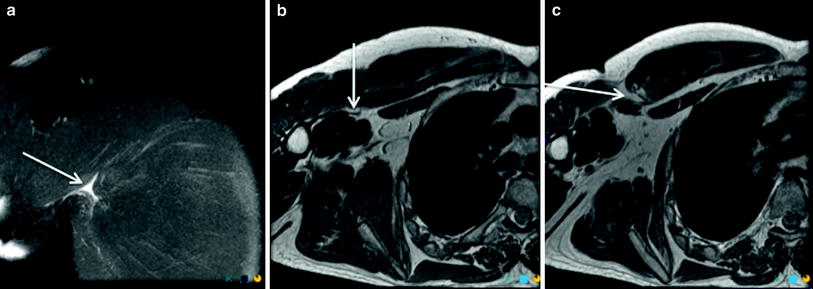
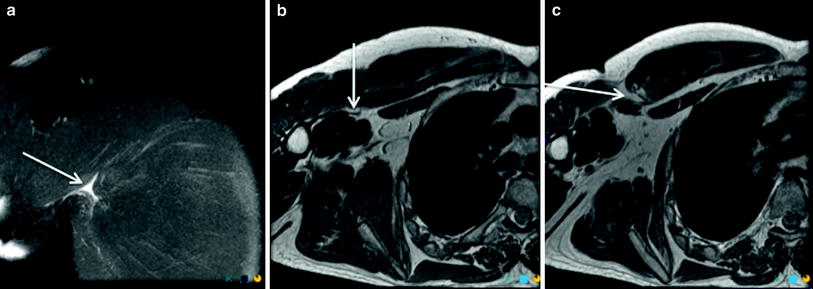
Fig. 9
Coronal proton density weighted MR image with fat saturation (a) and axial proton density weighted (b and c) images demonstrate a grade 3 muscle–tendon junction injury involving the sternal head of pectoralis major. The arrow in a denotes the small fluid collection at the site of tear. The arrow in b denotes the distal tendon edge, while the arrow in c shows the mild retraction of the muscle–tendon junction, with no discernible proximal tendon edge
Acute and subacute muscle tears are usually best visualised on longitudinal sequences (Linklater et al. 2010). In the subacute phase, immature scar tissue forming at the point of injury is generally of intermediate signal intensity tissue and often best appreciated on proton density sequences. When the scar tissue remodels, the signal intensity becomes increasingly more hypointense on all pulse sequences (Linklater et al. 2010) (Fig. 10).
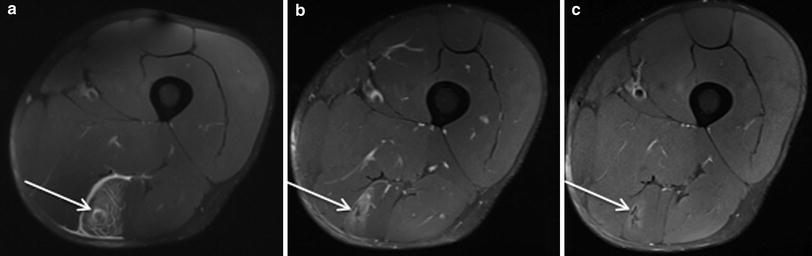
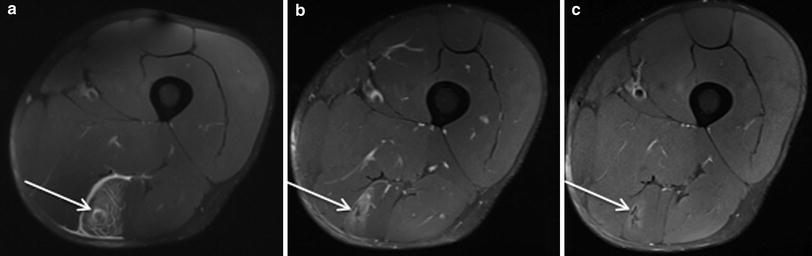
Fig. 10
Axial proton density weighted MR images with fat saturation (a–c) in a professional rugby league player. a Shows an acute semitendinosus muscle–tendon junction injury the day after the injury occurred, the arrow indicating a small focus of muscle fibre discontinuity, with surrounding feathery intramuscular oedema and thin layer of perifascial fluid. b 3 weeks post injury shows early scar formation and reduction in the extent of surrounding muscle oedema and resolution of the perifascial fluid. c 7 weeks post injury demonstrates maturation of scar tissue, with contraction of the scar and virtually complete resolution of the adjacent intramuscular oedema
Important findings to identify and document on MRI in the setting of muscle injury includes: site of injury, the presence/absence of fibre discontinuity, longitudinal extent and percentage cross-sectional oedema of muscle oedema, signal hyperintensity or frank fibre discontinuity within the intramuscular component of the tendon (Blankenbaker and Tuite 2010). Longer recovery times are associated with a larger volume of affected muscle, greater longitudinal extent and percentage cross-sectional area of muscle oedema, more cranially located injuries, and the presence of a haematoma (Askling et al. 2007; Connell et al. 2004; Gibbs et al. 2004; Malliaropoulos et al. 2010).
Healing muscle strains/tears demonstrate a reduction in the amount of oedema on fluid-sensitive sequences. Oedema post muscle injury may persist for weeks to months, even after return to sport and represents continuation of the healing process (Kijowski 2011). After the oedema has resolved, variable amounts of fatty atrophy and compensatory hypertrophy may be seen. T1 and T2 hypointense foci represent scar formation and residual blood degradation products (hemosiderin) (Blankenbaker and Tuite 2010; Linklater et al. 2010; Slavotinek 2010).
5.2.3 Muscle Contusion
The MRI appearance of a contusion may be similar to a muscle strain/tear, however may have a variable appearance depending on the age of the blood products. The typical appearance in mild contusion is a feathery appearance of diffuse muscle oedema on fluid sensitive sequences. More severe cases of contusion demonstrate a haematoma. T1-weighted sequences may be used to differentiate between haemorrhage/haematoma and oedema and to estimate the age of the haematoma (Hayashi et al. 2012) (Fig. 11).
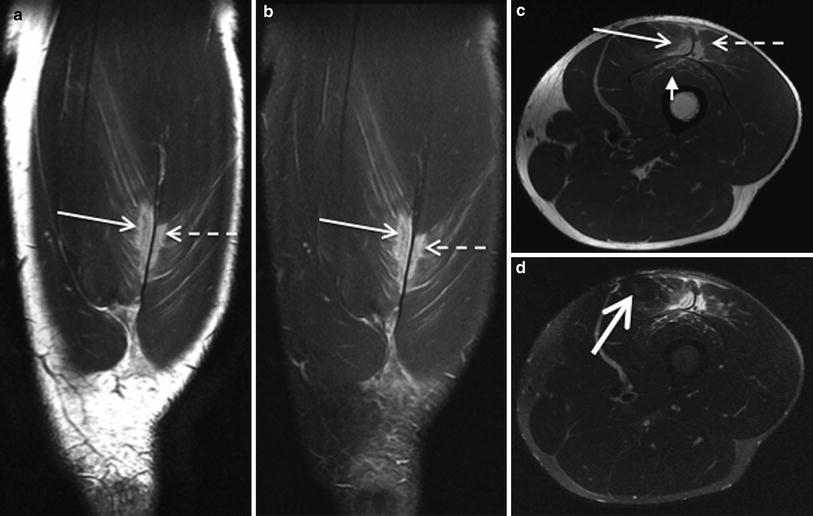
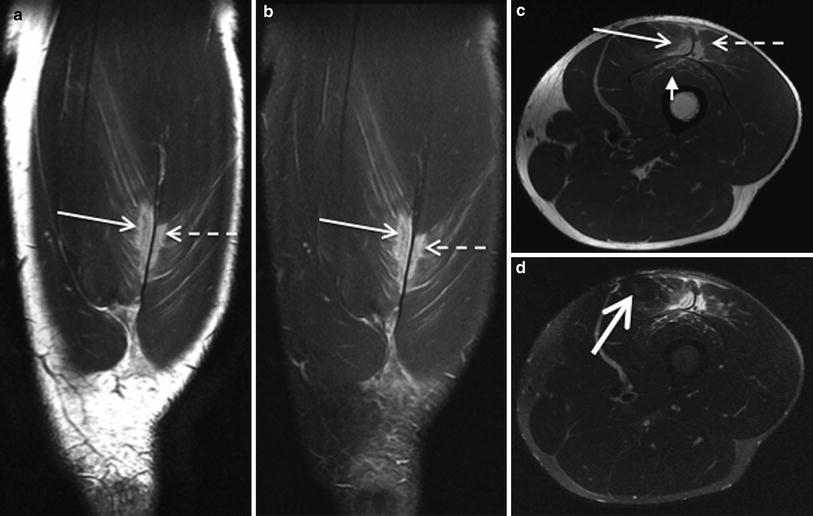
Fig. 11
Coronal proton density weighted MR images, with (a) and without fat saturation (b) and axial proton density (c) and STIR images (d) in a professional rugby league player, demonstrate a contusional injury involving the rectus femoris (arrow), vastus lateralis (dashed arrow) and vastus intermedius (short arrow) components of the quadriceps muscle group. Note the small haematoma at the epicentre of the injury, with somewhat geographic margins (thick arrow). The player returned to play at 2 weeks post injury
An acute haematoma is typically isointense to muscle on T1-weighted images and is hypointense on T2-weighted images. Methaemoglobin is the predominant component of a subacute haematoma (injury occurred less than 30 days prior), which is hyperintense relative to muscle on both T1-weighted and fluid-sensitive sequences. With further evolution of the blood products, a hypointense rim develops due to hemosiderin deposition and fibrosis and is visible on all pulse sequences (Blankenbaker and Tuite 2010) (Fig. 12). With evolution, a haematoma will reduce in size and will usually resolve within 6–8 weeks. An underlying cause should be sought if a haematoma persists longer than 6–8 weeks (Blankenbaker and Tuite 2010).
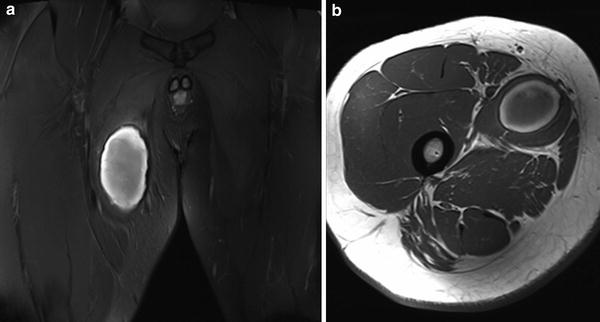
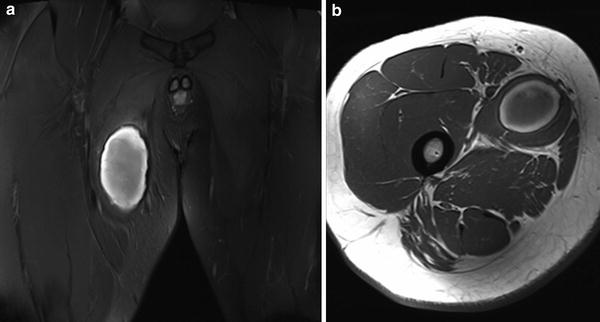
Fig. 12
Coronal proton density with fat saturation (a) and axial proton density weighted MR images (b) show a subacute haematoma within adductor longus following a muscle tear, with central hyperintensity and a hypointense peripheral rim
5.2.4 Delayed Onset Muscle Soreness
On MRI, recently exercised muscle demonstrates increased signal intensity on fluid-sensitive sequences secondary to an increase in intracellular water. This usually resolves within minutes of cessation of activity but may persist to some degree for several hours without symptoms (Fleckenstein et al. 1988).
The typical appearance of DOMS on MRI is difficult to differentiate from a muscle strain (grade 1). As stated previously, the differentiation of these two diagnoses may be the clinical history of the onset and duration of symptoms. There is “feathery” oedema within the muscle and perifascial oedema, which may persist for up to 80 days (Shellock et al. 1991). Although the areas of signal abnormality have been reported to correlate well with sites of structural injury, but poorly with sites of patient symptomatology, the literature in this area is sparse (Nurenberg et al. 1992) (Figs. 13, 14).
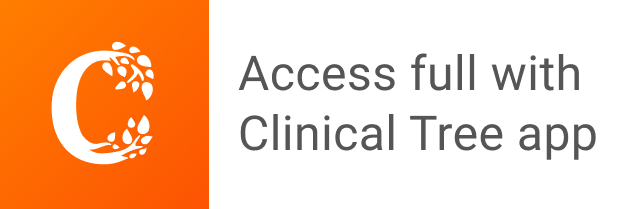