Fig. 1
Myopathic and neuropathic motor unit potentials (MUPs) on electromyography (EMG). a Short duration, polyphasic, low amplitude MUPs are seen in this quantitative EMG study of a patient with myopathy. b A tall, long duration MUP indicates chronic reinnervation in a patient with neuropathy
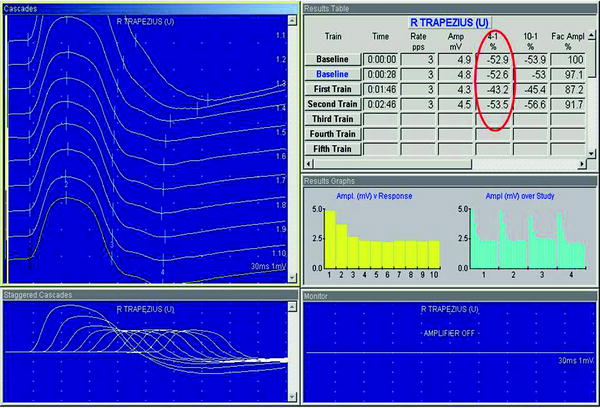
Fig. 2
Repetitive nerve stimulation (RNS) of the right trapezius in a patient with myasthenia gravis. In healthy controls, there should be no decline in the size of the nerve response. In this patient, the amplitude declines by 43–54 %, indicating a disorder of the neuromuscular junction
4.5 Nerve and Muscle Biopsy
Tissue biopsy is often necessary to provide an exact diagnosis (Fig. 3). Both muscle and nerve can be biopsied for histopathology and molecular studies. Muscle biopsy is indicated in patients with weakness, fatigue, myalgia, cramping, or persistently high CK. Muscle biopsy is typically performed following EDx testing, and, if possible, in a muscle not examined by EMG to avoid artifact from needle insertion. Some conditions, such as myasthenia gravis, are better diagnosed by NCS while other conditions (e.g., Duchenne muscular dystrophy), have such well-characterized genotypes that genetic testing is increasingly replacing muscle biopsy as the diagnostic test of choice. Muscle biopsy can be particularly helpful when evaluating patients with suspected systemic disorders, such as mitochondrial disorders as enzyme levels of mitochondrial function can be measured directly from muscle tissue. Additionally, systemic conditions, including amyloidosis (Chuquilin and Al-Lozi 2011) and sarcoidosis (Fujita et al. 2011; Le Roux et al. 2007), may involve the muscle, which allows for relatively accessible tissue for biopsy. Finally, molecular analysis, immunohistochemistry, and electron microscopy, allow for investigation of specific structural or immunologic elements that are either diagnostic or can direct specific genetic testing.
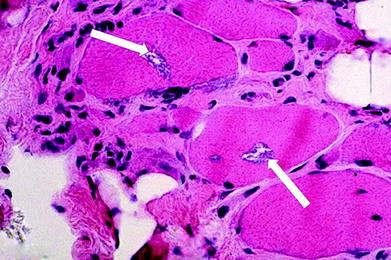
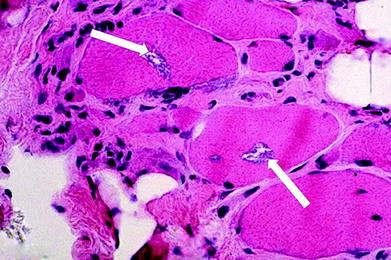
Fig. 3
Muscle biopsy in a patient with inclusion body myositis. In this patient, the characteristic rimmed vacuoles are seen (arrows). However, several biopsies are often needed to confirm the diagnosis secondary to the heterogeneous pattern of involvement
Like muscle, biopsy of nerve can be highly informative to the diagnosis and can impact treatment. Indications for nerve biopsy include asymmetric neuropathies, sensory loss in an easily accessible nerve, abnormal nerve conduction studies in an accessible nerve, or when the differential diagnosis suggests a potentially treatable disorder with high morbidity (e.g., vasculitis). Pathology may be present in only a portion of the nerve or muscle and may not affect all regions equally. Therefore, it is important to select a nerve or muscle that is clearly symptomatic. Unlike a muscle biopsy, nerve biopsy carries the potential for loss of function. Therefore, if possible, an affected sensory nerve at an easily accessible site is typically chosen for biopsy. If not, fascicular biopsy of an affected motor nerve may be performed in hopes of preventing any permanent loss of strength in affected muscles. Fascicular biopsies are becoming more common, as they can provide adequate diagnostic information without resulting in severe motor or sensory impairments (Campbell et al. 2009; Lyons 2009). Regardless of the technique utilized the patchy nature of some nerve and muscle diseases results in a significant number of false-negative biopsies. One study revealed that combined nerve and muscle biopsy yielded a diagnosis of vasculitis in only 50 % of cases (Collins et al. 2000) and it is well recognized that several muscle biopsies may be required to confirm the presence of inclusion body myositis (Dahlbom et al. 2002). Neuromuscular imaging could better inform the site selection for biopsy and therefore could improve the diagnostic yield of biopsies in these disorders.
4.6 Genetic Testing
A wide range of commercial genetic testing is now available. For some disorders, specifically Duchenne muscular dystrophy and spinal muscular atrophy (D’Amico et al. 2011), gene tests have largely replaced EDx testing and muscle biopsies. A directed, stepwise approach to testing is recommended in most cases. Batched testing is available to check for many limb-girdle muscular dystrophies (LGMD) or Charcot-Marie-Tooth disease. However, this broad approach to genetic testing is expensive and often includes conditions that are not only clinically distinct but also often exceedingly rare. The preceding elements of the evaluation (clinical history, physical examination, EDx results, and biopsy) should be combined to direct genetic testing toward a specific diagnosis. This minimizes the cost of testing and potential for misdiagnosis resulting from changes of unknown significance in genes that are clinically unrelated to the patient’s presentation. For the most unusual of cases, whole-exome sequencing is now available, but due to the high rate of polymorphisms of unknown significance, typically requires the assistance of a geneticist for accurate interpretation (Goh and Choi 2012).
5 Imaging of Muscle
While imaging of the arms and legs is commonly employed for evaluation of trauma or joint disease, and imaging of the brain and spine is commonly employed for evaluation of central nervous system disease, imaging in the evaluation of neuromuscular disease is not routinely performed. While imaging can be utilized to inform our understanding of neuromuscular disorders, it often identifies patterns of disease that can be clearly discerned from the history, physical exam, and EDx testing. However, with greater utilization and improved technical capabilities, it is increasingly apparent that in some neuromuscular disorders, imaging is uniquely informative and critical to the appropriate management of the patient. Imaging with MRI, US, and CT scans is most often performed. The following section is a brief introduction to the advantages and limitations of these different imaging modalities.
5.1 MRI of Skeletal Muscle
The signal characteristics of MRI are based upon the interaction between magnetic forces and the composition of tissues. Images are created from changes in the energy state of protons induced by magnetization. The time for return to equilibrium (the resting state) of protons is recorded. Fat has shorter T1 relaxation time relative to muscle and pure water or cerebrospinal fluid. Thus fat appears bright on T1 weighted images, while muscle appears intermediate and water appears dark (Fig. 4). T1 sequences are good for detecting fat deposition in chronic myopathies but are not sensitive for detecting increased intramuscular fluid that might be present in acute disease. T2 images display a different pattern. Here, muscle and tendons have a short T2 relaxation time, while water, cerebrospinal fluid, and edematous or inflamed tissues have a long relaxation time. Also, compared to muscle, fat takes longer to return to the resting state and thus appears brighter than normal muscle tissue. On T2 sequences, water will appear bright, making this the preferred sequence for visualizing acute or subacute muscle changes that may be related to edema or increased blood flow. In summary, the T1-weighted sequence is best for imaging fatty replacement of muscle seen in chronic neuromuscular disease and T2-weighted sequences are better for detecting acute or inflammatory changes of muscle.
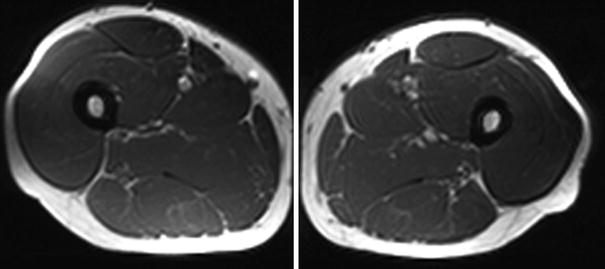
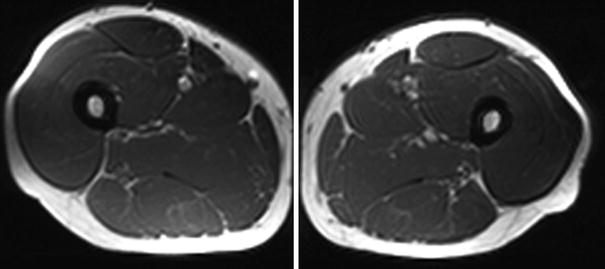
Fig. 4
Normal MRI of muscle in a healthy 32-year-old sports instructor. Axial T1-weighted images of the bilateral thighs reveals normal muscle without fatty infiltration (images courtesy of Prof. Dr. M. A. Weber, Heidelberg)
Short tau inversion recovery (STIR) sequences are also helpful in muscle imaging (Fig. 5). STIR uses a short inversion time to suppress fat signal. Thus the sequence will suppress signal from fat as well as any tissue with a relaxation time similar to that of fat, such as gadolinium (STIR sequences are not used with contrast agents for this reason) while allowing for signal from water. Some have advocated STIR as a replacement for T2 imaging for investigations of muscle injury (Evans et al. 1998). STIR sequences are faster than T2 sequences, and can therefore shorten the imaging time. This is extremely important for patients who have difficulty remaining immobile in the scanner and for whole body imaging studies, which are highly informative for determining patterns of muscle involvement (Schramm et al. 2008) and guiding muscle biopsy (Connor et al. 2007).
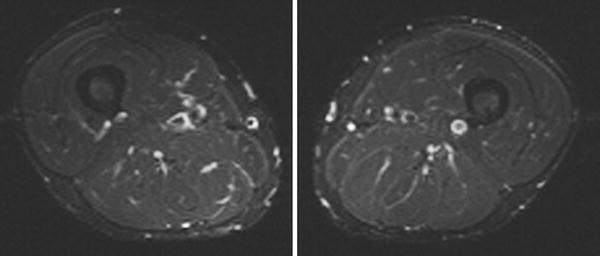
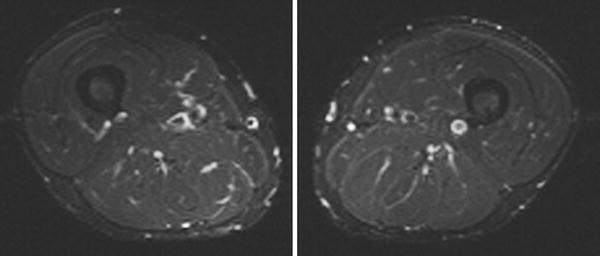
Fig. 5
Normal MRI of muscle (same subject as in Fig. 1.4). Axial STIR MRI of the bilateral thighs demonstrates no evidence of edema-like changes (images courtesy of Prof. Dr. M. A. Weber, Heidelberg)
Diffusion-weighted imaging (DWI) sequences are also used to examine muscle. DWI sequences provide information on fluid motion in tissues and are commonly employed to detect acute stroke. DWI can identify post-exercise muscle edema (Morvan 1995) and radiation injury to skeletal muscle (Baur and Reiser 2000). Quantification of edema-like changes in DWI sequences of inflammatory myopathies has potential applications for measuring disease progression and response to treatment (Qi et al. 2008).
Gadolinium, which shortens the T1 relaxation time, is not typically required to identify neuromuscular pathologies. Although the addition of gadolinium contrast can aid in the visualization of intramuscular pathology on T1 sequences, it does not appear to provide additive benefit in the evaluation of neuromuscular disease (Reimers et al. 1994a; Stiglbauer et al. 1993). In traumatic muscle injury, however, gadolinium may aid in diagnosis. A single prior study recommended the combination of STIR sequences and gadolinium contrast in cases of suspected muscle injury (el-Noueam et al. 1997). The authors reported four cases where both STIR and T2 sequences were negative for muscle strain, but the injury became apparent after the addition of contrast. For most purposes, the addition of contrast is limited to suspected cases of muscle tumors, abscess or other focal injury (Fig. 6).
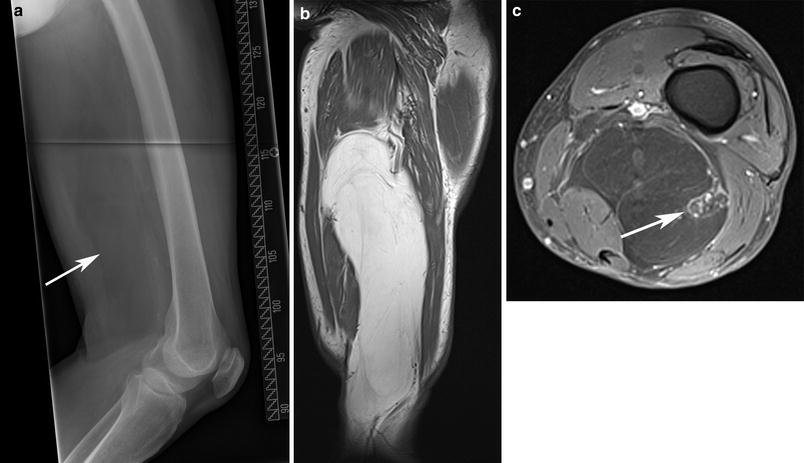
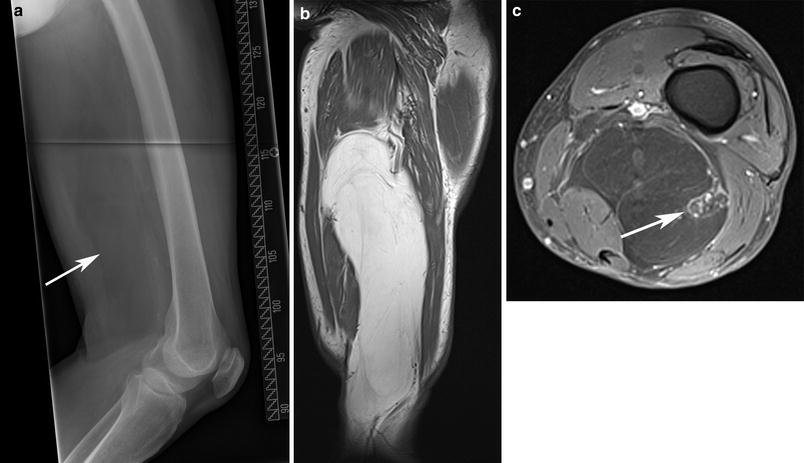
Fig. 6
Large (about 20 cm) lipoma of the thigh with encasement of the sciatic nerve in a 74-year-old man. The lipoma is seen as radiolucency in the projection radiograph (arrow in a), appears homogeneous on the T1-weighted sagittal MR image b and encases the sciatic nerve on the fat-saturated contrast enhanced, axial T1-weighted MR image (arrow in c). There are no areas of avid contrast enhancement and no thickened septae that are suspicious of a liposarcoma (images courtesy of Prof. Dr. M. A. Weber, Heidelberg)
MRI has many advantages for muscle imaging. MRI can image superficial and deep structures with equal efficacy and is not highly operator dependent. It is possible to image tissue from multiple and large areas of the body and is thus ideal for describing patterns of muscle involvement. It provides excellent differentiation between the muscle, fat, and adjacent tissues, including bone and tendon. New advances in MRI technology are now generating functional images that may provide additional information on muscle metabolism (see Chaps. 5–9 of this book). Quantitative measures are also potentially valuable for monitoring disease progression.
Despite these many advantages, MRI does have its limitations. It cannot provide dynamic images under standard circumstances, making it a less than ideal choice in select cases compared to ultrasonography as a real-time examination. Pediatric patients and other patients who are unable to remain still for the examination may require sedation, increasing the risk of the procedure. Finally, MRI carries a higher cost that either ultrasound or CT scans, a factor that cannot be overlooked in an increasingly cost-conscious health care environment. Analysis of the most cost-effective approaches to imaging as an ancillary test in neuromuscular disorders is needed.
5.2 Ultrasound Imaging
Ultrasound (US) imaging is based upon the interaction of sound waves with tissues that they encounter. The ultrasound transducer emits sound waves that travel through body tissues and these are reflected, refracted, scattered, or absorbed. Only the sound waves that are reflected back to the transducer are displayed on the resulting image. Objects like bone that reflect most sounds waves appear bright, or hyperechoic, on US imaging (Fig. 7). Structures that are fluid-filled transmit rather than reflect most of the sound waves and appear dark (hypoechoic).
Ultrasound carries many advantages in imaging muscle. It is less expensive than MRI or CT and there are no contraindications to testing. It can be performed without sedation in children and is painless. Clinicians can perform the test at the patient’s bedside, eliminating the need for additional referrals. US has high spatial resolution, making it ideal for visualizing small structures. Its major advantage over MRI or CT in muscle imaging is the ability to image and rapidly examine and compare multiple body regions (side to side comparisons, proximal to distal and arm to leg comparisons) and its ability to visualize the muscle in motion. This provides the opportunity to visualize fasciculations and other abnormal muscle movements. For comparison, in whole-body MRI, the patient is completely covered with coils (Figs. 8, 9) making this examination not suited for children or incompliant adults.
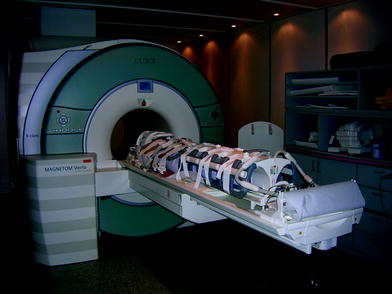
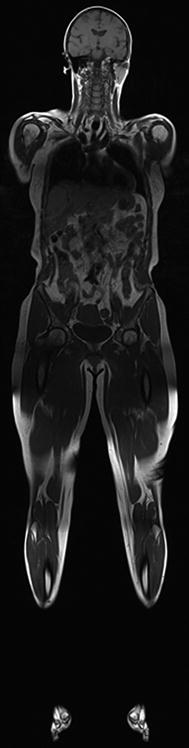
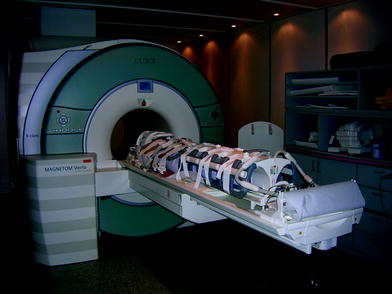
Fig. 8
Whole-body MRI. To obtain whole body MRI images, the entire body is covered in coils (image courtesy of Prof. Dr. M. A. Weber, Heidelberg)
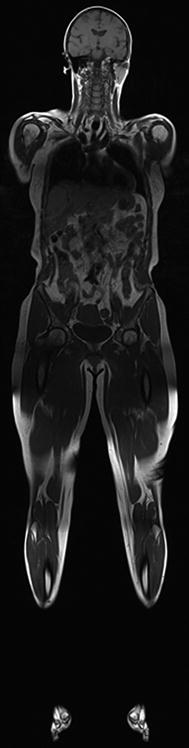
Fig. 9
Whole-body T1-weighted MR images of a healthy 50-year-old (image courtesy of Prof. Dr. M. A. Weber, Heidelberg)
Newer US technologies are expanding its diagnostic repertoire. Contrast-enhanced ultrasound refers to the use of microbubbles that are injected into the vascular system. High-energy ultrasound pulses create oscillations of these microbubbles, permitting the visualization of capillary perfusion within skeletal muscle (Amarteifio et al. 2011). This functional imaging technique can be performed at rest, during or after muscle contraction. Contrast-enhanced ultrasound will provide insight into microvascular disturbances that occur in muscular dystrophies and myositis (Fig. 10), aiding understanding of the underlying pathophysiology, while possibly serving as a biomarker for monitoring therapeutic response.
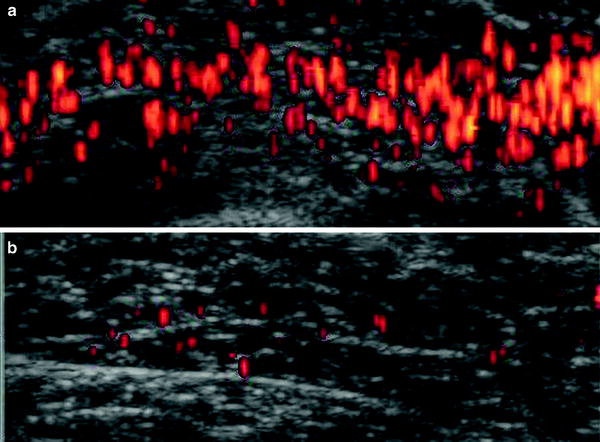
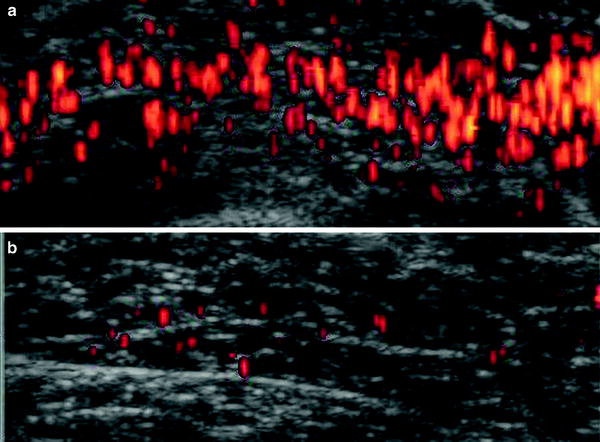
Fig. 10
Contrast-enhanced power doppler ultrasound reveals increased intramuscular blood flow in muscle of a woman with polymyositis a than in a woman with scleroderma b (modified from Weber et al. (2007))
Ultrasound elastography is another promising tool that is currently in development. Elastography permits measurement of tissue rigidity or stiffness and is already being utilized in the assessment of liver disease and breast masses. For muscle, its use remains investigational, as researchers continue to devise reliable and accurate means of quantifying muscle stiffness. Different methods include manual compression and measuring tissue strain (Chino et al. 2012; Palmeri et al. 2011). Newer methods of US elastography include delivery of a high frequency pulse followed by measurement of movement in the tissue region of interest (known as acoustic radiation force imaging). Elastography techniques may permit detection of structural changes that alter the stiffness of skeletal muscle. As many myopathies are characterized by increased intramuscular fat and fibrous tissue, elastography is a promising technique for detecting the pathology associated with muscle disease.
Ultrasound is not always the optimal choice for imaging. Deeper muscles cannot be well depicted by most structures. Bone impedes the view of any structure that lies behind it, limiting views of some muscles. Obesity can impede optimal visualization of proximal or deep muscles. Acute pathology that results in increased edema like signal on MRI may be undetectable or subtle on ultrasound. Severe chronic pathology, with replacement of muscle with fat and fibrosis, may prevent identification of muscle borders or intramuscular architecture on ultrasound.
5.3 Computed Tomography and Radiographs
In a nutshell, computed tomography (CT) scans use X-ray images taken from multiple directions that are then compounded to make a cross-sectional image. CT scans provide axial images with high resolution and good detail of the soft tissues. Newer technologies are available and CT now has multiplanar imaging capabilities (Kuo and Carrino 2007). The use of CT imaging for evaluation of muscle is limited. While it is able to distinguish bones, tendons, ligaments, and other structures associated with muscle, it is not very sensitive in detecting inflammation or edema. It can detect fatty changes, along with abnormal mineralization of the soft tissues (Calo et al. 1986; Swash et al. 1995).
With increasing use of MRI, CT is less often used for the assessment of neuromuscular disorders. It exposes patients to ionizing radiation and is not the optimal test for detecting the edema and/or inflammation in muscle. The same may be said for plain radiographs, which can identify calcinosis of soft tissues, but does not provide detail on the muscle itself (Kuo and Carrino 2007). CT scans are, however, much faster to obtain than MRI and offer improved contrast resolution and imaging of deep tissue compared to ultrasound.
6 Muscle Imaging in Neuromuscular Disorders
6.1 Overview
Imaging of injured and diseased muscle can reveal a diverse array of alterations. Abnormal muscle typically shows changes in signal characteristics, and can also show changes in size, shape, movement, metabolism, and blood flow. The choice of imaging modality, typically either MRI or US, affects which abnormal property of muscle will be best appreciated. For instance, while MRI yields superior imaging of deep tissue and identification of signal changes from increased water content compared to US, sonography is superior for identifying abnormal muscle movements. The differential diagnosis, and the suspected underlying pathology, must drive the decision of when and how to image a patient with a suspected neuromuscular disorder.
Unique to neuromuscular disorders is the relative importance of the pattern and distribution of muscle pathology within and between muscle groups (Costa et al. 2012). Muscle pathology, when identified, should be described as focal, patchy, or diffuse. Focal changes or space occupying lesions suggest most often a traumatic or infectious etiology including herniation, strain, hematoma, and infection, but rarely also a tumor of the muscle or muscle sheath (see “MRI in Muscle Tumors and Tumors of Fasciae and Tendon Sheaths” of this book). Myositis ossificans, a focal area of heterotopic bone formation within the muscle, is a sign of chronicity and can occur following myositis, trauma, or bleeding (Tyler and Saifuddin 2010). Sheets of heterotopic intramuscular bone suggest a diagnosis of myositis ossificans progressiva, a rare inherited disorder characterized by recurrent, transient focal enlargements of muscle, and progressive ossification of connective tissues. Patchy or diffuse involvement of muscle can be seen with inherited or acquired myopathies and infectious or inflammatory myositis. Myonecrosis or ischemic myositis, sometimes occurring in diabetes mellitus, can cause patchy involvement of a single muscle or muscle groups. Diffuse, symmetric, and proximal muscle involvement suggest a myopathic process, as opposed to neuropathy which affects the distal most muscles of the legs and, in advanced cases, arms. It is important to note that inherited myopathies can show highly selective and variably stereotyped patterns of muscle involvement, as discussed in following sections.
Muscle shape and size can be quantified using many imaging modalities, and can assist in the identification of disease (Heckmatt et al. 1988a; Hudash et al. 1985). In asymmetric disorders, comparison to the less or unaffected limb can be helpful in identifying the presence and degree of abnormality in muscle size. With more diffuse disorders, thickness measurements should be compared to appropriate reference values. It is important to note that muscle thickness varies with age and gender, and pathologic processes often do not affect all muscles equally (Ota et al. 2012; Arts et al. 2007). Exercise and body habitus can also affect muscle size. These factors must be considered when referencing normative values.
In neuromuscular disorders, muscle can be normal in size, atrophic, hypertrophic, or pseudohypertophic. Atrophy is histologically defined by small muscle fiber size and results in reduced muscle bulk. In isolation, atrophy of muscle is nonspecific and can occur from disease of the nerve or muscle or from disuse. The combination of atrophy and abnormal muscle signal makes disuse much less likely. Denervation is more likely to result in muscle atrophy than myopathy. In many myopathies, muscle bulk is relatively preserved despite abnormal muscle signal and, often, weakness. Hypertrophy of muscle can occur from compensation due to weakness of neighboring muscle groups or in response to disease. Muscle can also appear hypertrophic following tendon tear, as the retracted muscle belly will thicken. Myotonia congenita, acromegaly, or space occupying lesions in the muscle (e.g., infection, tumor, or inflammation) can also increase muscle size. In the acute phase of myositis, mild muscle hypertrophy can be seen, presumably related to increased blood flow and/or water content or from inflammation within the muscle (Pillen et al. 2008b). Pseudohypertrophy, as opposed to true hypertrophy, occurs when increased intramuscular fat leads to enlarged muscle bulk. Thus, the thickened appearing muscle in pseudohypertrophy stems not from true muscle hypertrophy but from infiltration and replacement with fat (De Beuckeleer et al. 1999; Lovitt et al. 2006). This is classically seen in the calves of patients with Duchenne muscular dystrophy, but is not specific for this disorder (Reimers et al. 1996a).
Changes in muscle signal properties are perhaps the most common abnormalities noted in neuromuscular disorders. MRI has an advantage to CT scan and US as MRI sequences can best detect and differentiate increased fat from edema-like changes (May et al. 2000). Increased T1 signal in muscle suggests increased fat content of muscle, as often seen in muscular dystrophy, chronic myopathies, or severe, long-standing denervation. Early fatty replacement in muscle appears as patchy or marbled increased T1 signal, whereas diffuse or complete fatty infiltration of muscle reflects more chronic, longstanding, or severe disease (Fig. 11). Alternative etiologies of increased T1 signal include methemoglobin, highly proteinaceous fluid, early calcinosis, scar formation, or mass occupying lesions. Increased T2 signal is often detected in myopathic or denervated muscle. The pathogenic etiology of the increased T2 signal is unknown and may result from inflammation, increased blood flow, or increased water content. Again, the pattern of muscle involvement affects the differential diagnosis (Costa et al. 2012). Focal increased T2 signal within the muscle typically results from trauma. Diffusely increased T2 signal limited to a single muscle suggests severe strain or denervation. Increased T2 signal in an isolated group of adjacent muscles suggests infectious myositis, deep vein thrombosis, compartment syndrome, or, when sharply demarcated, radiation injury. Increased T2 signal in symmetric nonadjacent muscles suggests a myopathy and is particularly informative in the identification and management of inflammatory myopathies, as T2 signal changes appear early and revert with successful treatment, although resolution of changes in MRI with treatment may lag clinical changes by months. Decreased T1 or T2 signal can results from calcifications and fibrosis (Reimers 2000).
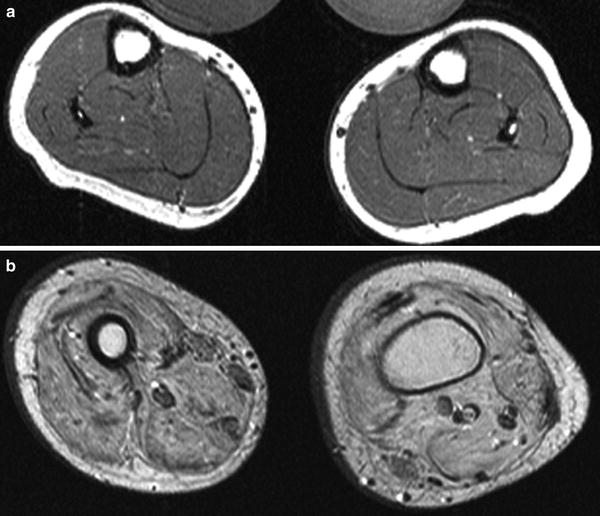
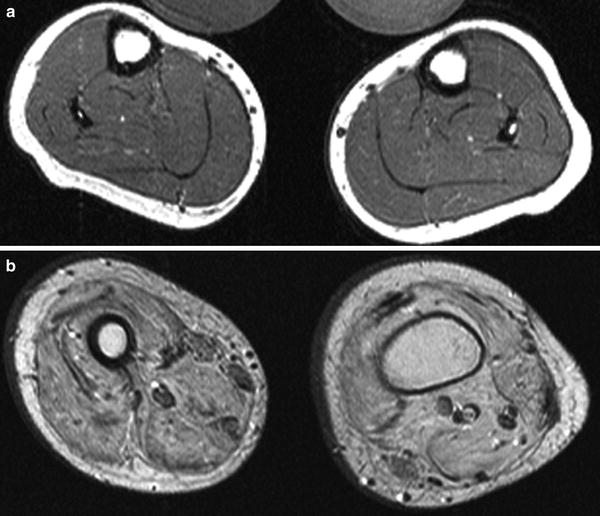
Fig. 11
T1-weighted MR images from a 7-year-old (a) and 14-year-old (b) boys with Duchenne muscular dystrophy demonstrate increased fat content within the muscl e, worse in b with more severe pathology (images courtesy of Prof. Dr. M. A. Weber, Heidelberg)
Imaging with ultrasound and CT also can be helpful in assessing suspected neuromuscular disorders. Increased fatty or fibrotic tissue is readily detected with CT or US scan but may not detect edema-like pathology seen only on T2 or STIR MR sequences. Muscle echogenicity on US increases with greater amounts of intramuscular fat and fibrosis (Heckmatt et al. 1989; Pillen et al. 2009). In the authors’ experience, US of inflammatory myopathies shows increased echogenicity without the attenuation seen in disorders characterized by fatty replacement of muscle. Thus, a highly echogenic muscle with a well-preserved bone reflection suggests an inflammatory myopathy over a muscular dystrophy.
One advantage of ultrasound over other imaging modalities is that evaluation in real time permits the identification of pathologic muscle movement, particularly fasciculations. Fasciculations are more readily detected with ultrasound than EMG or the clinical exam (Reimers et al. 1996b). Fasciculations are fast, random, nonrhythmic movements in a portion of the muscle that deform its shape but typically do not cause movement of the joint. Care must be taken to assure the imaged muscle is fully relaxed. In healthy individuals, fasciculations often are limited to muscles in the distal leg and are known to increase in frequency with age (Fermont et al. 2010). Other factors, including caffeine consumption, certain medications (e.g. pyridostigmine), and recent exercise may temporarily increase the frequency as well (Fermont et al. 2010; Simon and Kiernan 2013). Frequent and/or widespread fasciculations can occur in benign cramp-fasciculation syndrome or following denervation and are of particular diagnostic significance in amyotrophic lateral sclerosis (Misawa et al. 2011; de Carvalho and Swash 2013). Denervation is the likely etiology when fasciculations are seen in conjunction with weakness, reduced muscle thickness and increased signal intensity. However, denervation cannot be excluded in an otherwise normal appearing muscle with fasciculations. It is the authors’ practice to obtain electromyography to further evaluate for denervation when fasciculations are detected, particularly when they are not restricted to the distal leg and more frequent than 1–2 every 10 seconds. Fibrillation potentials can also cause abnormal muscle movement detected with ultrasound. Previously identified only by electromyography, the tiny movements generated by profuse fibrillation potentials can also be detected using ultrasound, although their detection is neither sensitive nor specific (van Alfen et al. 2011). Muscle tremor may also be recorded, but in the authors’ experience this adds little to the clinical examination.
6.2 Denervation
Denervation of muscle results in changes in the shape, size, and composition of muscle. These changes can be detected using both ultrasound and MRI (Kullmer et al. 1998). On MRI, denervated muscles is characterized by increased T2 signal, but the etiology is of this change is unknown. Although often attributed to edema, there is no supporting histopathologic evidence. Increased blood flow and extracellular fluid are two alternative mechanisms that could explain T2 signal change (Kamath et al. 2008; Wessig et al. 2004). Increased T2 signal in muscle is not specific for denervation. A recent study of 23 patients with forearm trauma demonstrated transient, increased signal in nondenervated hand musculature (Viddeleer et al. 2011). This study emphasizes the nonspecific nature and uncertain origin of fluid weighted signal change on muscle MRI following trauma or denervation. Despite this lack of understanding, clear patterns of evolving signal change on US and MRI follow denervation. These have been described in both experimental and, less commonly, clinical settings and when applied correctly, could be beneficial for patient care.
Following experimental denervation, increased relaxation time of fluid weighted sequences such as T2 and STIR may occur within 2 days of injury. Over time, replacement of chronically denervated muscle with fat and fibrotic tissue results in hyperintensity on T1-weighted sequences. With reinnervation, T2 signal changes begin to resolve and ultimately disappear. Wessig and colleagues found that that T2 relaxation time of muscle showed a high temporal concordance with electrodiagnostic findings of denervation and reinnervation after experimental denervation (Wessig et al. 2004). T2 signal acutely increased following denervation and then returned to normal as regeneration and voluntary activity of denervated muscle reappeared. Increases in T2 signal closely paralleled increases in the total capillary area identified on histologic examination of the affected muscle. Following reinnervation, both capillary size and T2 signal decreased, suggesting that increased blood volume is a strong contributor to T2 signal changes following denervation.
Similar to MRI, muscle ultrasound can be used to evaluate the presence and extent of denervation. High resolution ultrasound detects nerve transection immediately after injury and can influence clinical decision making (Cartwright et al. 2007; Padua et al. 2013). Changes in muscle are also seen on ultrasound following denervation, but not as quickly as with MRI. Increased echogeneity and loss of normal intramuscular architecture can appear approximately 2 weeks following acute nerve lesions. Muscle echointensity increases along with the severity and chronicity of denervation (Gunreben and Bogdahn 1991). As with MRI, the onset of sonographic changes parallels electrodiagnostic evidence of denervation. In contrast with MRI, ultrasound can detect abnormal movements in denervated muscle with fasciculations seen most prominently. In conjunction with electrodiagnostic studies, this dynamic assessment may improve the diagnostic sensitivity for lower MNDs, including amyotrophic lateral sclerosis (Misawa et al. 2011).
Both MRI and ultrasound can monitor changes in muscle size following denervation (Kullmer et al. 1998). While muscle atrophy is most commonly associated with denervation, both muscle hypertrophy and pseudohypertrophy may also occur (Petersilge et al. 1995). Alterations in muscle size following denervation are of particular prognostic interest. Zaidman et al. performed a study using ultrasound in 51 infants with newborn brachial plexus palsy. They compared each infant’s affected limb with the unaffected opposite side and found that muscle thickness, but not muscle signal, correlated with function and increased with functional recovery overtime (Zaidman et al. 2012). Relative to the uninjured limb, muscle hypertrophy (mean increase 17 %) was also seen more frequently in children with moderately impaired strength. In contrast, in muscles with severely impaired strength, atrophy was seen, being 15 % thinner than the normal side. Another recent study of muscle cross-sectional area in 31 infants with obstetrical brachial plexus lesions used MRI. In general, both elbow flexors and extensors had reduced areas as compared to the unaffected side. However, this was not true in each individual. The authors demonstrated that muscle hypertrophy occurred in 17 of 31 patients following denervation, but found no correlation between muscle size and function (Ruoff et al. 2012).
A study by Viddeleer et al. (2011) provides evidence for the potential value of monitoring muscle signal change following denervation. Twenty-three patients with traumatic transection of the median or ulnar nerve, who had also undergone nerve repair, were enrolled. MRI of the affected hand was performed, including short inversion time inversion recovery (STIR) sequences. Patients underwent imaging at 1, 3, 6, 9 and 12 months after nerve repair. The authors found that STIR MRI could differentiate between denervated and reinnervated muscles up to 1 year after repair. Normalization of signal intensity correlated with good functional recovery, while persistently elevated signal was seen in those with poor hand function at 12 months. Prospective studies are required to determine the diagnostic and prognostic value of serial changes in muscle size and signal on patient care and recovery following denervation (Berciano et al. 2012).
6.3 Myopathy
Muscle imaging, particularly with MRI, can be incorporated into the clinical assessment of myopathies. Appropriately directed imaging can augment the diagnostic and clinical assessment of patients with myositis, muscular dystrophies, and other myopathies. Imaging is particularly helpful in ascertaining involvement of muscles that cannot be easily assessed on physical or electrodiagnostic testing. Whole-body MRI techniques allow for relatively rapid image acquisition not limited to the upper or lower limbs (Quijano-Roy et al. 2012) and for identification of patterns of muscle involvement that inform the differential diagnosis (reviewed by Pillen et al. (2008a) and Mercuri et al. (2005a), see also Chap. 4 of this book). Although additional investigation is needed, quantitative imaging techniques in MRI or US could potentially be used to monitor disease progression.
Algorithms for deducing specific genetic etiologies using patterns of radiologically determined muscle involvement have been described (Wattjes et al. 2010). The success of these strategies is variable and depends upon the specific type of myopathy. One study compared visual analysis of MRI patterns in 83 patients with rigid spine muscular dystrophy caused by SEPN1 mutations, Bethlem and Ulrich congenital muscular dystrophy (COL6A1, COL6A1, and COL6A1), Emery–Dreifuss muscular dystrophy (LMNA mutations) and limb-girdle muscular dystrophy 2A (CAPN3). These were compared with a control group of 25 other patients with other forms of muscular dystrophy. All subjects underwent conventional T1-weighted spin echo MRI. The authors found that compared to other myopathies, these disorders have highly specific (0.96) and sensitive (0.90) patterns of myopathology. Rigid spine muscular dystrophy and LGMD2A were most easily identified, but there was high interobserver agreement for all scans (Mercuri et al. 2010). Another study of other myopathies and using CT scan was not as promising. Four evaluators reviewed the imaging of 188 patients with seven different types of limb-girdle muscular dystrophy and found that the sensitivity of patterns of myopathology was high in Bethlem myopathy (90 %) and Becker muscular dystrophy (BMD) (91 %), but not for other limb-girdle muscular dystrophies (Ten Dam et al. 2012). This is likely in part because some genotypes share overlapping features. Additionally, the study had poor overall interobserver agreement (κ = 0.27), raising concern for reproducibility of the results. This highlights the need for prospective studies to determine the diagnostic utility of imaging in the evaluation of suspected hereditary myopathies.
Although these studies raise questions regarding routine imaging of patients with suspected myopathies, in particular instances, it is clear that muscle imaging can reveal patterns of abnormalities or involvement of muscles not easily detected on the physical exam. For instance, a hereditary form of inclusion body myopathy is typically characterized clinically as having “quadriceps sparing”, because knee extension strength is relatively preserved compared to sporadic inclusion body myositis. However, US of six patients with hereditary “quadriceps sparing” inclusion body myositis and homozygous glucosamine (UDP-N-acetyl)-2-epimerase/N-acetylmannosamine kinase (GNE) mutations revealed severe, selective involvement of the rectus femoris with relatively sparing of the vastus medialis, vastus lateralis, and vastus intermedius (Adler et al. 2008). Thus, imaging can detect abnormalities within muscles or groups of muscles that may not be apparent clinically.
The following sections highlight examples of neuromuscular disorders in which imaging has been shown to impact the diagnostic work-up or to identify muscle involvement that could aid in diagnosis or monitoring of disease progression.
6.4 Immune and Inflammatory Myopathies
Immune and inflammatory myopathies (IIM) are a group of heterogeneous myopathies that are classically subdivided based on clinicopathologic features into dermatomyositis (juvenile and adult), polymyositis, and inclusion body myositis. The heterogeneous clinical and pathologic features of these disorders complicate their categorization and an alternate classification based on myopathologic features has recently been proposed (Pestronk 2011). Most imaging studies rely on the traditional classification conventions. To reflect the most widely accepted nomenclature and avoid confusion, we will organize this section according to the classic terminology with the realization that disparate disorders may be termed “polymyositis” despite having variable clinical and pathologic features.
Dermatomyositis and polymyositis are immune-mediated disorders that result in muscle inflammation and weakness. Muscle weakness is typically proximal, symmetric, and progressive. Patients describe functional impairment in rising from chairs, stair climbing, or lifting objects overhead. In severe cases, dysphagia and respiratory weakness may occur. Myalgias are reported in a considerable number of patients. Creatine kinase levels are typically elevated; when absent, an elevated aldolase can suggest the diagnosis (Casciola-Rosen et al. 2012).
Dermatomyositis is associated with several cutaneous manifestations (Bohan and Peter 1975a, b; Khan and Christopher-Stine 2011). These include a violaceous, erythematous rash about the periorbital region (heliotrope), symmetrically about the neck and shoulders (shawl sign), or a linear erythema over the limb extensor regions or joints. Gottron’s papules, flat topped violet colored papules over the extensor joints of the fingers are highly common. Scaling and hyperkeratosis of the hands (mechanic’s hands) can also occur. Cutaneous manifestations of the disease can occur concurrently with or precede the onset of weakness. These skin findings can occur in the absence of identifiable muscle symptoms, a process termed amyopathic dermatomyositis.
Electromyography readily identifies inflammatory myopathies, but is not particularly specific or helpful in differentiating subtypes of diseases. Abnormal spontaneous activity (fibrillations, positive sharp waves, and complex repetitive discharges) is accompanied by short duration, polyphasic motor unit potentials, and early recruitment. Unfortunately, these changes may be patchy with some areas of muscle appearing entirely normal. Myopathologic findings display the heterogeneous nature of these disorders and include myovasculopathy in juvenile more than adult dermatomyositis, perifascicular inflammation, and myofiber necrosis and regeneration (Pestronk 2011). Serum antibody markers (e.g. anti-Jo-1, anti-SRP, etc.) also aid in the diagnosis of inflammatory myopathies, but are not present in all patients (Casciola-Rosen et al. 2012).
MRI investigations of the IMMs have been well documented (Del Grande et al. 2011). Muscle MRI demonstrates increased edema-like changes within and surrounding the muscle (Fig. 12), along with fatty infiltration and calcification (Reimers et al. 1994b; Hernandez et al. 1990, 1993). Increased edema-like changes on MRI are more prominent than increased signal on fat sensitive sequences, especially in dermatomyositis. Edema-like changes are more commonly seen during the acute phase of illness, when they correlate with the degree of weakness and inflammatory infiltrate seen on biopsy (Reimers et al. 1994b; Tomasova Studynkova et al. 2007). In some cases, MRI abnormalities were detected more frequently than elevated creatine kinase levels and revealed muscle disease in patients with apparent amyopathic dermatomyositis (Reimers et al. 1994b; Lam et al. 1999; Stonecipher et al. 1994). Given the patchy distribution of muscle pathology in IIMs, pre-biopsy MRI can improve the diagnostic yield of muscle biopsy. In one study of MRI and IIM (Schweitzer and Fort 1995), a false-negative biopsy occurred in only 1 of 14 patients randomized to MRI before biopsy compared to 5 of 11 who did not have an MRI. Those randomized to MRI also had lower total average medical costs.
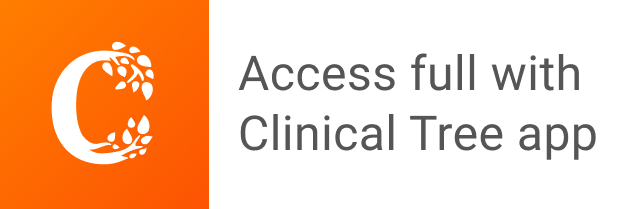