Fig. 1
Structure of a peripheral nerve (type: myelinated axon)
Pathophysiology and Classification System
Several categorizations of peripheral neuropathies exist, including one that is based on the underlying etiology. It distinguishes between entrapment and nonentrapment neuropathies. Nerve entrapment syndromes at the upper extremity typically affect the median, radial and ulnar nerves at anatomic locations where the nerve courses through nerve fibro-osseous or fibromuscular tunnels or penetrates a muscle. In cases of nerve thickening, narrowing of the tunnel or hypertrophy of the muscles, the nerve might be entrapped. Nonentrapment neuropathies include all other etiologies such as traumatic nerve injuries, inflammatory conditions, polyneuropathies and mass lesions [1, 3].
Other categorizations distinguish systemic diseases from local as well as functional diseases. Systemic diseases include ischemia, vasculitis, toxic, endocrine and metabolic disorders (e.g., diabetes), motorsensory neuropathies, amyloidosis, hyperlipidemia, multifocal motor neuropathies and inflammatory neuropathies. MRI is not expected to establish these diagnosis but may help to confirm the clinical suspicion, to show the abnormality of the involved nerves and muscles, and to document the distribution/pattern of the neuropathy [3]. Local diseases include all local abnormalities such as masses, nerve injuries, entrapment neuropathies, infections and adhesive changes following surgery. MRI has a major role in these conditions as it adds morphological information to clinical information. Imaging can show the exact localization of the lesion, and detect the underlying nerve or other pathology [3]. Functional diseases such as traction or compression mononeuritis due to habitual leg crossing or repeated typing/exercise are difficult to evaluate by MRI, as in most cases only indirect signs are visible. Dynamic assessment of peripheral nerves by the means of MRI is often not conclusive. However, ultrasound can then be an important tool to evaluate, for example, functional compressive neuropathies, since it allows dynamic or functional nerve assessment during exercise.
Peripheral nerve injuries are typically classified according to the Seddon and Sunderland classification system. In this classification system three different types of nerve injury are described, namely: neuropraxia, axonotmesis and neurotmesis [3].
Neuropraxia is the least severe type of injury. It is characterized by a predominant loss of motor function due to a temporary block of the nerve conduction. Usually there is also some degree of loss of sensory function. Neuropraxia often is associated with blunt trauma where the myelin sheaths are injured. Wallerian degeneration (a process in which the part of the axon separated from the neuron’s cell body degenerates distal to the injury) does not occur in neuropraxia. The electromyogram is usually negative. This type of injury is relatively mild and MRI of the peripheral nerve may show some abnormal increased nerve signal on fat suppressed T2-weighted magnetic resonance (MR) images, but typically no muscle denervation changes are seen [3]. Usually, there is no disruption, effacement or enlargement of the involved nerves.
Axonotmesis is a more severe type of injury and leads to axonal injury and Wallerian degeneration distal to the site of injury. Loss of both sensory and motor function occurs. Axonotmesis is characterized by axonal discontinuity and damage to the covering endoneurium. However, it is important to remember that the remaining connective tissue framework of the nerve (epineurium and perineurium) is usually preserved. As a consequence, axonotmesis injuries have the potential to recover spontaneously because the surrounding myelin sheaths remain intact. Typical clinical findings include decreased or absent evoked muscle and sensory action potentials. Electrodiagnostic tests are therefore useful for distinguishing the lower grade of injury (neuropraxia) from the more severe grade of injury (axonotmesis) [3]. Typical MRI findings include signs of muscle denervation as well as increased T2 signal intensity of the nerve on fat-suppressed images. Morphological changes can also be detected. The latter include fascicular enlargement, disruption and/or nerve effacement. Axonal regeneration occurs slowly at a rate of approximately 1 mm per day from the proximal to the distal nerve segment [3]. With advancing nerve regeneration, normalization of the abnormal T2-hyper — intensity of the nerve can be seen on MR images. The typical time frame for meaningful functional recovery is several weeks to months.
Neurotmesis is the most severe type of nerve injury, resulting from severe contusion, crush, stretch or lacerations [3]. Axonal lesions, with complete disruption of the surrounding perineurium and epineurial layers, cause complete loss of motor, sensory (and autonomic) function. In most cases, neurotmetic injuries cannot be differentiated from axonotmetic injures by means of electrodiagnosis or clinical examination. They usually do not recover because of a complete disruption of axons and connective tissue, as this makes spontaneous functional regrowth virtually impossible [3, 4]. Imaging has the potential to show a complete nerve transection in acute cases or neuroma formation in chronic cases. Although nerve conspicuity is somewhat hampered in the acute phase by hemorrhage, imaging is usually able to differentiate neurotmetic from axonotmetic injures. Longstanding muscle denervation often results in severe fatty muscle infiltration and atrophy, which severely limits the success of surgery.
Other classification systems, such as those from Sunderland et al. and Mackinnon et al., exist but discussion is beyond the scope of this syllabus.
Peripheral nerves affected by entrapment syndromes are typically compressed along their course at predisposed anatomic locations, i.e., tunnels. The floor of these tunnels usually consists of bone, whereas the roof is usually made up of focal transverse thickenings of the deep fascia, retinaculae and muscle aponeurosis [1, 3–6]. Pathophysiology of entrapment syndrome is different from other acute nerve injuries, as the development of compressive neuropathy depends on the pressure within these tunnels and the fact that nerves typically can withstand only little pressure. At more than 15 mmHg, the venous supply to the nerves is increasingly hampered, and at over 40 mmHg the arterial blood supply is affected. Irreversible structural nerve damage begins at pressures of around 80 mmHg [3]. Typical MR findings of compressed nerves include increased signal intensity on T2-weighted MR images. Recent studies have demonstrated that the large myelinated axons at the outer portions of the nerve are most affected. This relative sparing of the fibers in the central portion of the nerve supports the theory that the primary mechanism of injury is shear forces; therefore, ischemia appears to be a secondary mechanism [3]. In the early stages, symptoms can be intermittent or even disappear spontaneously. As the disease progresses, fibrotic nerve changes can occur causing further nerve impingement. In chronic cases, severe axonal degeneration and myelin loss are common histological findings. Clinically, there is a permanent loss of nerve function. Long-standing disease causes fatty infiltration and atrophy of the denervated muscles. The abnormal T2 hyperintensity of the nerve, along with proximal enlargement, angulation and displacement, are characteristic findings of nerve entrapment. Regional muscle denervation changes on MR images support the diagnosis of neuropathy.
Technical Considerations and Normal Appearance of Nerves*
MR neurography is a tissue-specific imaging technique optimized for evaluating peripheral nerves, their internal fascicular morphology (see above), alteration in neural signal and caliber, and associated space occupying and other compressive lesions [7]. Three-dimensional (3D) imaging is of critical importance in tracing the course of peripheral nerves, in identifying points of compression or disruption, and for preoperative planning. In general, MR neurography can be either T2 based or diffusion based. Diffusion- based MR imaging, especially diffusion tensor imaging, allows functional assessment of the nerves, but as yet remains a novel technique, with specific hardware and software requirements in an attempt to enhance the otherwise low signal-to-noise ratio (SNR) from these small nerves, limiting its application in routine clinical practice.
The Magnet
The strength of the magnet is an important consideration, with impact on both image quality and speed of acquisition [8]. In general, there is superior performance of MR neurography at 3 Tesla (3T) compared with 1.5T. In our institutions, we perform the technique using 3T platforms only. In fact, it is the advent of high Tesla imaging and its widespread availability that has facilitated the development of state of the art MR neurography and made it a reality in daily clinical practice. Compared with 1.5T MRI, 3T MRI provides increased (nearly double) SNR, which in part is related to improved coil design, better gradient performance and wider bandwidths. This translates into higher spatial resolution and thinner slice sections with improved fluid conspicuity, as well as superior contrast-to-noise ratio, which improves anatomic characterization and lesion detection [2]. Increased conspicuity of fluid and more uniform fat-suppression techniques result in better depiction of fascicular appearance of the nerves. There is also less inhomogeneity of the magnetic field. More robust hardware facilitates the application of multiple radiofrequency saturation pulses required for adequate vascular suppression. Furthermore, the application of parallel imaging allows faster acquisition times. It is difficult to produce quality T2-weighted 3D images on lower field strength magnets because of time and hardware limitations. 3D gradient echo sequences often have to be employed, and images thus generated are frequently nonisotropic with poor SNR and soft tissue contrast, and are prone to susceptibility artefacts [2]. In contrast, high-quality isotropic 3D proton density and T2-weighted images can be acquired with relative ease and speed at 3T, and serve as an invaluable adjunct to two dimensional (2D) images.
MR Protocol
T1-Weighted Imaging
High-resolution T1-weighted imaging is excellent for depicting normal anatomy of the peripheral nerves and surrounding structures. Thin sections (maximum slice thickness of 4 mm) are necessary for adequate resolution of anatomic detail and fascicular morphology. Peripheral nerves appear as linear T1 hypointense structures, following an expected anatomical distribution. Differentiation from adjacent vessels is often possible, especially with the larger nerves, with arteries appearing as flow voids and veins appearing T1 hyperintense due to inflow phenomenon [9]. With larger nerves or at higher resolution imaging, the individual fascicles may be resolved [7]. Peripheral nerves are outlined by T1 hyperintense perineural fat, with a characteristic reverse tram track appearance of alternating T1 hyperintense and T1 hypointense signal that increases their conspicuity. Infiltration of the perineural fat and soft tissues is often best depicted on T1-weighted imaging. The presence of muscle fatty replacement in the setting of long-standing denervation is also seen to best advantage on this imaging sequence.
T2-Weighted Imaging
Pathological changes within the nerve are seen to best advantage on T2-weighted images [10]. In addition, many mass lesions and pathological processes that commonly result in neural compression (e.g., paralabral and ganglion cysts, peripheral nerve sheath tumors, fluid-filled bursae) are best characterized and are most conspicuous on T2- weighted images. With standard fast spin-echo, (non fat suppressed) T2-weighted imaging, it is difficult to discern abnormal increased T2 signal from perineural and intraneural fat, therefore fat-suppressed T2-weighted imaging is the optimal sequence for detecting neural pathology. This imaging sequence is also most sensitive for early changes of muscle denervation signal alterations. However, there are drawbacks to fat-suppressed T2-weighted imaging, with more artefacts from hyper — intense vascular structures and partial volume averaging [11]. Vascular structures, which typically accompany the nerve, appear hyperintense and can be confused with neural signal abnormality or perineural edema.
Dedicated MR neurography utilizes increased T2 weighting with thinner slices, to increase conspicuity of T2 signal change and achieve higher spatial resolution [11]. Maximizing the conspicuity of increased T2 signal in nerves is achieved in three ways: (1) utilizing sequences with long echo times (90–130 ms), (2) applying radiofrequency saturation pulses to suppress signal from adjacent vessels, and (3) utilizing frequency selective or adiabatic inversion recovery imaging type fat suppression [10]. This is best achieved at higher field strength, emphasizing the importance of technological advances in the development of high-resolution neural imaging. This results in increased T2 weighting while minimizing spurious signal from adjacent vessels and fat, and it is more sensitive for demonstrating neural signal abnormality [12]. Newer techniques employed in 3D imaging, namely steady state free precession and diffusion techniques, result in superior suppression of vascular signal in T2-weighted sequences, particularly when imaging the extremities [13].
3D Imaging
Isotropic 3D imaging is an essential component of state of the art MR neurography. Nerves often follow oblique courses and are not seen to best advantage on standard axial, coronal and sagittal imaging planes. 3D multiplanar reconstructed (MPR), curved planar reconstructed (CPR) and maximal intensity projected (MIP) images greatly facilitate the visualization of nerves and are particularly useful for preoperative planning. Decreased magic angle artefact and partial volume averaging on 3D imaging allow for more accurate depiction of pathology. Furthermore, caliber and signal change in nerves, which may be subtle or attributed to volume averaging on axial imaging, are seen to better advantage in the longitudinal plane, allowing better assessment of the true extent of the abnormality. Certain pathologies, such as plexiform neurofibromata, particularly lend themselves to 3D imaging for depiction of the entire disease burden in one image (Fig. 1). Compression of peripheral nerves by disc protrusions, space occupying lesions and anatomical fibroosseous tunnels is also more accurately delineated on 3D imaging. Focal interruption of a nerve can be particularly challenging to demonstrate on axial imaging, and 3D reconstructions may prove to be crucial in this scenario. Variations in muscular volume and anatomy may also be better assessed on 3D imaging.
A number of techniques can be employed in 3D imaging. SPACE (sampling perfection with application optimized contrasts by using different flip angle evolutions; Siemens, Erlangen, Germany) sequence is an isotropic single slab acquisition, mainly spin-echo type sequence, which allows MPR, CPR and MIP reconstructed images to map out the peripheral nerves and associated pathology in the longitudinal plane. This may be obtained in SPAIR (spectral adiabatic inversion recovery) or STIR (short tau inversion recovery sequence) contrasts, thus providing T2 weighting and optimizing sensitivity for detecting neural pathology. STIR imaging is preferred for imaging of the lumbosacral and brachial plexus due to superior fat suppression, while SPAIR imaging is preferred for imaging of the extremities. More recent developments include the 3D diffusion-weighted reversed fast imaging with steady state free precession (3D DW-PSIF) sequence, which is a heavily T2-weighted technique, combining selective suppression of vascular flow signal with multiplanar capabilities. The PSIF sequence holds great promise, particularly in the imaging of the extremities. Attempts to suppress vascular flow signal with the application of saturation bands usually fail in peripheral locations, due to the slow or in-plane flow within the peripheral vessels, or due to the variable oblique courses of peripheral neurovascular bundles. PSIF, because of its sensitivity to flow-related dephasing of the transverse magnetization, causes vessels in the imaging field of view to lose their signal intensity. Vascular signal suppression is also enhanced by the small diffusion moment applied to this sequence [13].
The Field of View
Large field of view is most commonly utilized in pelvic neurography protocols. This occurs at the expense of resolution, but allows side to side comparison and evaluation of multiple nerve distributions on a single study. In our experience, the nerves most commonly affected in pelvic neuropathy can be reliably demonstrated when a dedicated MR neurography protocol is performed. In larger patients, and when a particularly large field of view is required, 3D STIR-SPACE might be preferable to 3D SPAIR-SPACE imaging given the superior and more robust fat suppression.
Pitfalls and Technical Limitations
Magic angle effect, a well-described phenomenon in imaging of tendons, also occurs when imaging peripheral nerves. This results in spurious increased signal when the nerve lies in a plane 55 degrees to the main vector of the magnet. However, unlike tendons, this effect may persist in nerves, even at longer TE (>66 ms), and therefore higher TEs must be utilized to overcome it [14, 15]. Traditionally, the interpreting radiologist was advised to be particularly aware of this phenomenon when reporting increased intraneural T2 signal in MR neurography studies; however, more recently published literature concludes that magic angle effect is in reality a rare source of false-positive interpretation on MR neurography, particularly in nerves that run parallel to the main vector of the magnet [3].
Despite the use of suppressive radiofrequency pulses, hyperintense vascular signal is often present on MR neurography examinations, especially at high TE. This is a particular source of confusion in smaller nerves, when it occurs in the accompanying vascular bundle, and might be misinterpreted as neural or perineural signal abnormality. Inhomogeneous fat suppression is another potential source of error, which is particularly true in the pelvis, given the large field of view and the high number of patients who have hip or lumbar instrumentation, which further degrades the local magnetic field, thus worsening fat suppression. Increased susceptibility and chemical shift artefact, also seen at 3T, can be counteracted by shortening the echo time, performing parallel imaging and increasing the bandwidth. Nonetheless, 1.5T imaging might be preferable when evaluating nerves in close proximity to a metal prosthesis. Specific absorption rate limits are reached earlier at 3T compared with 1.5T, as there is increased energy deposition for radiofrequency excitation at 3T. However, this is usually balanced by faster image acquisition and shorter examination time and does not usually pose a clinical problem. Other potential drawbacks of 3D imaging include longer imaging times, and time spent producing and interpreting multiplanar reformatted images.
Commonly Imaged Pathologies at the Upper Extremity
MR neurography is often used for the evaluation of entrapment syndromes at the upper extremity. These can affect the median and radial nerves, as well as the ulnar nerve. Detailed knowledge of the anatomy of the individual nerves is important for image interpretation, and a good text book showing the innervated muscles helps to indirectly localize the site of the nerve lesion based on the pattern of involved muscles in cases where direct visualization of the pathology is not feasible.
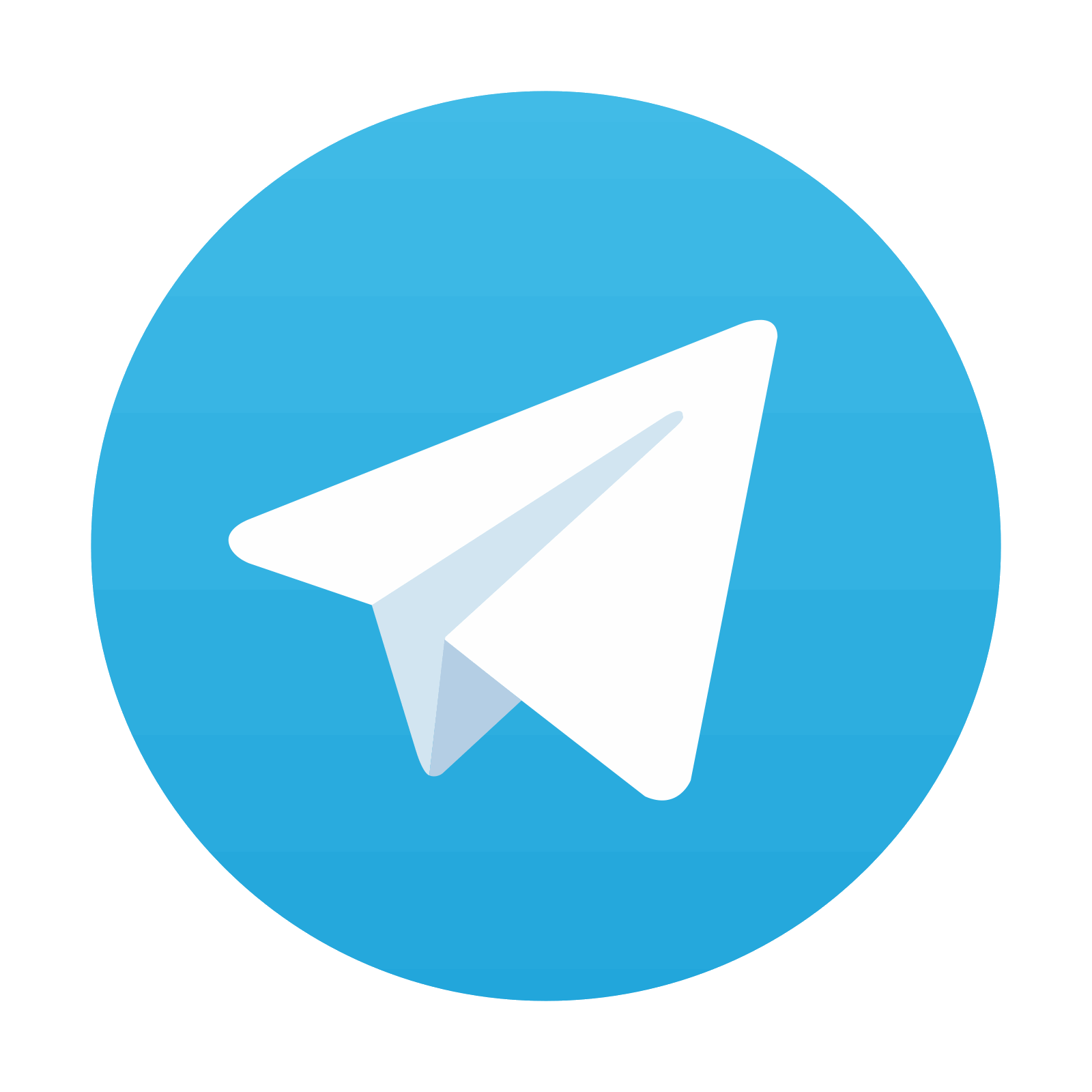
Stay updated, free articles. Join our Telegram channel
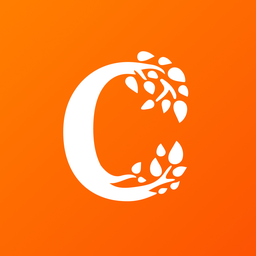
Full access? Get Clinical Tree
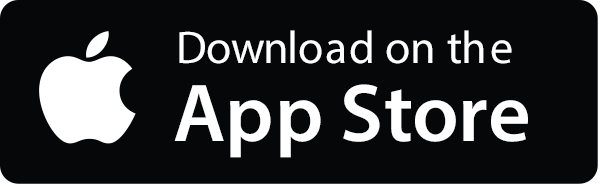
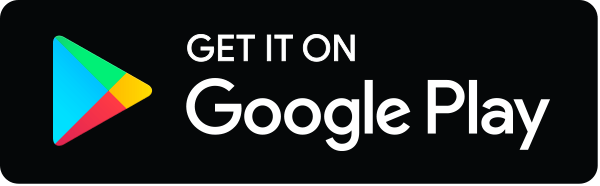