(1)
Faculty of Medicine, Department, of Oncology and Medical Physics Unit, McGill University, Montreal, Québec, Canada
Abstract
Chapter 14 consists of 12 problems spread over 6 sections that deal with practical aspects of particle accelerators in medicine. Many types of particle accelerators were built for nuclear physics and particle physics research and most of them have also found some use in medicine, mainly but not solely for treatment of cancer. Two categories of particle accelerator are known: electrostatic and cyclic.
The best-known examples of electrostatic accelerator are the x-ray tube and the neutron generator. Three types of x-ray tube (Crookes tube, Coolidge tube, field emission carbon nanotube) are covered in this chapter. Cyclic accelerators are divided into two categories: linear and circular. Many types of circular accelerator have been designed for research purposes and most are also used in medicine, such as betatron, microtron, cyclotron, and synchrotron.
Of all cyclic accelerators, the linear accelerator (linac) is by far the most important and most widely used accelerator in medicine because of its versatility and compact design. Modern radiotherapy achieved its successes as a result of the advances that were introduced during the past few years in the linear accelerator technology and computerization, making the dose delivery extremely sophisticated and heavily dependent on skills of the radiotherapy team consisting of radiation oncologist, medical physicist, radiation dosimetrist, and treatment technologist.
Section 14.1 concentrates on basic characteristics of particle accelerators and Sect. 14.2 deals with practical use of x rays. Practical considerations in production of x rays are covered in Sect. 14.3, while Sect. 14.4 consists of several problems on traditional sources of x rays. Section 14.5 concentrates on circular accelerators and covers the betatron, microtron, yclotron, synchrotron, and synchrotron light source; all machines that have been or are used in medicine. The chapter concludes with a large section covering various practical issues related to linear accelerators (linacs) used in radiotherapy for generation of megavoltage xray and electron beams.
Chapter 14 consists of 12 problems spread over 6 sections that deal with practical aspects of particle accelerators in medicine. Many types of particle accelerators were built for nuclear physics and particle physics research and most of them have also found some use in medicine, mainly but not solely for treatment of cancer. Two categories of particle accelerator are known: electrostatic and cyclic.
The best-known examples of electrostatic accelerator are the x-ray tube and the neutron generator. Three types of x-ray tube (Crookes tube, Coolidge tube, field emission carbon nanotube) are covered in this chapter. Cyclic accelerators are divided into two categories: linear and circular. Many types of circular accelerator have been designed for research purposes and most are also used in medicine, such as betatron, microtron, cyclotron, and synchrotron.
Of all cyclic accelerators, the linear accelerator (linac) is by far the most important and most widely used accelerator in medicine because of its versatility and compact design. Modern radiotherapy achieved its successes as a result of the advances that were introduced during the past few years in the linear accelerator technology and computerization, making the dose delivery extremely sophisticated and heavily dependent on skills of the radiotherapy team consisting of radiation oncologist, medical physicist, radiation dosimetrist, and treatment technologist.
Section 14.1 concentrates on basic characteristics of particle accelerators and Sect. 14.2 deals with practical use of x rays. Practical considerations in production of x rays are covered in Sect. 14.3, while Sect. 14.4 consists of several problems on traditional sources of x rays. Section 14.5 concentrates on circular accelerators and covers the betatron, microtron, yclotron, synchrotron, and synchrotron light source; all machines that have been or are used in medicine. The chapter concludes with a large section covering various practical issues related to linear accelerators (linacs) used in radiotherapy for generation of megavoltage xray and electron beams.
14.1 Basic Characteristics of Particle Accelerators
14.1.Q1 (289)
Numerous types of accelerator have been built for basic research in nuclear physics and high-energy physics and most of them have been adapted for at least some limited use in radiotherapy.
(a)
State the three basic physical conditions that must be met for particle acceleration in an accelerator.
(b)
State the two major classes of particle accelerator and briefly explain the main characteristics of each class.
(c)
Provide a table that lists: (i) Most important accelerators in physics, (ii) Their inventor, if known, (iii) Year of invention, (iv) Particles accelerated by the accelerator, (v) Is or was the particular accelerator used in radiotherapy?
(d)
Summarize the list of most important accelerators in physics in a classification diagram format.
SOLUTION:
(a) Irrespective of the accelerator type, three basic physical conditions must be met for particle acceleration:
The various types of particle accelerators differ in the way they produce the accelerating electric field and in how the field acts on the charged particles to be accelerated in the machine.
(1)
Particle to be accelerated must be charged (either positively or negatively).
(2)
The accelerator must provide electric field for particle acceleration.
(3)
The electric field used for particle acceleration must be provided in the direction of particle motion.
(b) As far as the accelerating electric field is concerned, there are two main classes of accelerator: (1) electrostatic and (2) cyclic.
(1) In electrostatic accelerators the particles are accelerated by applying an electrostatic electric field through a voltage difference, constant in time, whose value fixes the value of the final kinetic energy of the accelerated particle. Since the electrostatic fields are conservative, the kinetic energy that the particle can gain depends only on the point of departure and point of arrival and, hence, cannot be larger than the potential energy corresponding to the maximum voltage drop existing in the machine. Kinetic energy that an electrostatic accelerator can reach is limited by the discharges that occur between the high voltage terminal and the walls of the accelerator chamber when the voltage drop exceeds a certain critical value (typically 1 MV).
(2) The electric fields used in cyclic accelerators are variable and non-conservative, associated with a variable magnetic field and resulting in some close paths along which the kinetic energy gained by the particle differs from zero. If the particle is made to follow such a closed path many times over, one obtains a process of gradual acceleration that is not limited to the maximum voltage drop existing in the accelerator. Thus, the final kinetic energy of the particle is obtained by submitting the charged particle to the same, relatively small, potential difference a large number of times, each cycle adding a small amount of energy to total kinetic energy of the particle.
Cyclic accelerators fall into two main categories: linear and circular, depending on particle’s trajectory during the acceleration. In a linear accelerator the particle undergoes rectilinear motion, while in a circular accelerator the particle’s trajectory is circular. All cyclic accelerators except for the linear accelerator fall into the category of circular accelerator.
Examples of electrostatic accelerators used in medicine are: superficial and orthovoltage x-ray machines and neutron generators. In the past, Van de Graaff accelerators have been used for megavoltage radiotherapy, however, their use was discontinued with the advent of first the betatron and then the linear accelerator. For medical use, the best-known example of a cyclic accelerator is the linear accelerator (linac); all other examples fall into the circular accelerator category and are the microtron, betatron, cyclotron, and synchrotron.
(c) A list of most important particle accelerators in physics is given in Table 14.1.
Table 14.1
Some characteristics of most important particle accelerators in physics
Electrostatic accelerators | Inventor(s) | Year of invention | Particles accelerated | Used in radiotherapy |
---|---|---|---|---|
X-ray machine | William Crookes | ∼1872 | Electrons | YES |
William Coolidge | 1913 | |||
Neutron generator | – | – | Deuteron | YES |
Van de Graaff generator | Van de Graaff | 1929 | Electrons Protons | YES |
Cockroft-Walton generator | John D. Cockroft | 1932 | Protons | No |
Ernest T.S. Walton | ||||
Cyclic accelerators | ||||
Linear accelerator | – | – | Electrons | YES |
Protons | ||||
Betatron | Donald Kerst | 1940 | Electrons | YES |
Microtron | Vladimir Veksler | 1950 | Electrons | YES |
Cyclotron | Leo Szilard | 1932 | Protons | YES |
Ernest Lawrence | Heavier ions | |||
Synchrocyclotron | Edwin McMillan | 1952 | Protons | NO |
Heavier ions | ||||
Synchrotron | Vladimir Veksler | ∼1944 | Electrons | YES |
Edwin McMillan | Protons |
(d) Charged particle accelerators are divided into two main categories: electrostatic and cyclic and each of the two categories is subdivided further into several distinct subgroups depending on the specific technique they use for particle acceleration. The classification diagram for most important particle accelerators is given in Fig. 14.1.
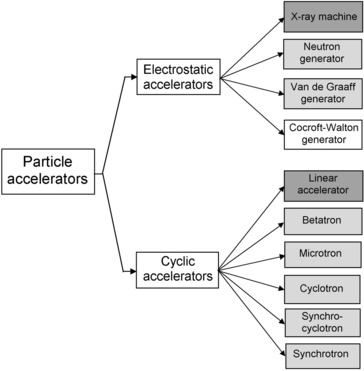
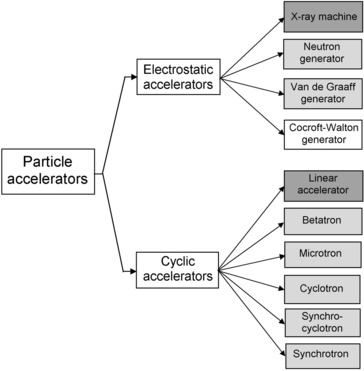
Fig. 14.1
Classification diagram of particle accelerators of importance in physics and medical physics. Accelerators used in medicine in addition to physics are shown with grey background; the two most common machines (x-ray machine and linear accelerator) are shown in darker grey color
14.2 Practical Use of X Rays
14.2.Q1 (290)
The serendipitous discovery of x rays by Röntgen in 1895 ushered in the era of modern physics and spawned two new medical specialties and a new physics specialty.
(a)
Name and briefly describe the three new specialties resulting from the discovery of x rays.
(b)
Two other radiation physics discoveries soon after Röntgen’s discovery of x rays in 1895 also made a significant contribution to science in general and medicine in particular. Name and briefly discuss the two discoveries.
(c)
X rays are not only used in science and medicine, they are also heavily used in industry and in many other aspects of modern life. List and briefly discuss at least five areas of x ray use in industry and modern life.
(d)
Three areas of x-ray physics: (1) x-ray crystallography, (2) x-ray spectroscopy, and (3) x-ray astronomy became scientific specialties in their own right. Briefly discuss the three scientific specialties.
SOLUTION:
(a) The usefulness of x rays for medical applications in diagnosis and treatment of human disease became apparent within a few weeks after Wilhelm Röntgen’s discovery of x rays in 1895. First came the use of x rays for imaging of diseased organs, for locating foreign objects imbedded in tissues (gun shot wounds) and for identifying broken bones, eventually leading to the medical specialty of diagnostic radiology.
Concurrently with the development of the x-ray imaging modality, attempts were made to use x rays for treatment of disease, eventually leading to the medical specialty of radiotherapy, also called therapeutic radiology or radiation oncology.
From their early beginnings both diagnostic radiology as well as radiation oncology relied heavily on physicists for routine use of radiation and for developments of new techniques and equipment. The involvement of physicists in the two new medical specialties eventually lead to a specialty of physics referred to as medical physics. During the past two decades medical physics has undergone a tremendous evolution, progressing from a branch of applied science on the fringes of physics into an important mainstream discipline that can now be placed on equal footing with other more traditional branches of physics such as nuclear physics, particle physics, and condensed matter physics.
Initially, most technological advances in medical use of ionizing radiation were related to:
During the past two decades, on the other hand, most developments in radiation medicine were related to integration of computers in imaging, development of digital diagnostic imaging techniques, and incorporation of computers into therapeutic dose delivery with high-energy linear accelerators. Radiation dosimetry and treatment planning have also undergone tremendous advances in recent years: from development of new absolute and relative dosimetry techniques to improved theoretical understanding of basic radiation interactions with human tissues, and to the introduction of Monte Carlo techniques in the determination of dose distributions resulting from penetration of ionizing radiation into tissue.
(1)
Improvements in efficient x-ray beam delivery.
(2)
Development of analog imaging techniques.
(3)
Optimization of image quality with concurrent minimization of delivered dose.
(4)
Increase in beam energies for radiotherapy.
(b) Two physics discoveries, important not only for modern physics but also for radiation medicine, followed soon after Röntgen’s discovery of x rays. In 1896 Henri Becquerel discovered natural radioactivity; the discovery eventually leading to the physics specialties of nuclear physics and high-energy physics and a subspecialty of diagnostic radiology referred to as nuclear medicine. In 1898 Marie Sklodowska-Curie and Pierre Curie discovered radium and the discovery lead to applications of sealed radionuclides in treatment of malignant disease referred to as brachytherapy.
(c) X rays for industrial use are produced by x-ray machines, linear accelerators (also called linacs), and betatrons, depending on the x-ray energy required. Superficial and orthovoltage x rays originate in x-ray machines, x-rays in the megavoltage range of energies are produced by linear accelerators and betatrons Industrial use of x rays covers a wide variety of purposes dealing with safety and quality assurance issues, such as:
(d) In addition to stimulating new specialties in medicine and physics, as mentioned in (a), and to some extent in industry, as mentioned in (c), x rays also enabled new developments in physics, chemistry, and astronomy, such as: (1) x-ray crystallography, (2) x-ray spectroscopy, and (3) x-ray astronomy.
(1)
Inspection of luggage, shoes, mail, cargo containers, etc.
(2)
Nondestructive testing and inspection of welds, cast metals, parts of automobiles and airplanes, iron reinforcement bars, cracks and pipes inside concrete structures.
(3)
Food irradiators for sterilization and pest control.
(4)
Ionizing radiation based sterilizers of surgical equipment and blood irradiators.
(5)
Small animal irradiators for radiobiological experiments.
(1) X-ray crystallography is a study of crystal structures through the use of x-ray diffraction techniques. X rays are very suitable for this purpose because their wavelength in the 10 keV to 100 keV energy range is of the order of typical crystalline lattice separations. An x-ray beam striking a crystalline lattice is scattered by the spatial distribution of atomic electrons and the imaged diffraction pattern provides information on the atomic or molecular structure of the crystalline sample.
In 1912 Max von Laue established the wave nature of x rays and predicted that crystals exhibit diffraction phenomena. Soon thereafter, William H. Bragg and William L. Bragg analyzed the crystalline structure of sodium chloride, derived the Bragg relationship 2dsinϕ=mλ linking the lattice spacing d with x-ray wavelength λ, and laid the foundation for x-ray crystallography. The crystal lattice of a sample acts as a diffraction grating and the interaction of x rays with the atomic electrons creates a diffraction pattern which is related, through a Fourier transform, to the electron spectral distribution in the sample under investigation.
Instrumentation for x-ray diffraction studies consists of a mono-energetic x-ray source, a device to hold and rotate the crystal, and a detector suitable for measuring the positions and intensities of the diffraction pattern. Mono-energetic x rays are obtained by special filtration of x rays produced either by an x-ray tube or from an electron synchrotron storage ring. The basic principles of modern x-ray crystallography are essentially the same as those enunciated almost 100 years ago by von Laue and the Braggs; however, the technique received a tremendous boost by incorporation of computer technology after the 1970s, increasing significantly the accuracy and speed of the technique.
(2) X-ray spectroscopy is an analytical technique for determination of elemental composition of solid or liquid samples in many fields, such as material science, environmental science, geology, biology, forensic science, and archaeometry (archaeological science). The technique is divided into three related categories: the most common of them is the x-ray absorption spectrometry (also called x-ray fluorescence spectrometry), and the other two are x-ray photoelectron spectrometry and Auger spectrometry. All three techniques rely on creation of vacancies in atomic shells of the various elements in the sample under study as well as on an analysis of the effects that accompany the creation of vacancies (e.g., emission of photoelectron, emission of characteristic line spectrum, and emission of Auger electron). Like other practical emission spectroscopic methods, x-ray spectroscopy consists of three steps:
While x-ray spectroscopy was initially used to further the understanding of x-ray absorption and emission spectra from various elements, its role now is reversed and it is used as a non-destructive analytical tool for the purpose of chemical analysis of samples of unknown composition.
(i)
Excitation of atoms in the sample to produce fluorescence emission lines (or photoelectrons or Auger electrons) characteristic of the elements in the sample.
(ii)
Measurement of intensity and energy of the emitted characteristic lines (or electrons).
(iii)
Conversion of measured data to concentration or mass with the nanogram range reached with standard spectrometers.
(3) X-ray astronomy is a relatively new branch of astronomy dealing with the study of x-ray emission from celestial objects, such as neutron stars, pulsars, and black holes. The specialty was born in 1962 when Italian-American astronomer Riccardo Giacconi discovered a cosmic x-ray source in the form of a compact star located in the constellation of Scorpius. For this discovery Giacconi received the 2002 Nobel Prize in Physics.
Since the x-rays emitted by celestial objects have relatively low energies of the order of a few kiloelectron volts, they cannot penetrate through the Earth’s atmosphere to reach the surface of the Earth. Thus, to study these celestial rays, detectors must be taken above the Earth’s atmosphere. Methods used to achieve this involve mounting x-ray detectors on rockets, balloons, or satellites. The x-ray detectors used for this purpose are either special charge-coupled devices (CCDs) or microcalorimeters.
14.3 Practical Considerations in Production of X Rays
14.3.Q1 (291)
In principle, all charged particles can emit radiation under certain conditions. In practice, however, the choice of charged particles that can produce measurable amounts of radiation of interest in medical physics, medicine, and industry is limited to light charged particles (electrons and positrons). It is well known that light charged particles interacting with an absorber (target) emit characteristic radiation and bremsstrahlung photons. However, it is less known that, when light charged particles interact with a target, other types of radiation may also be produced, depending on specific conditions related to the particle interaction with the target atoms.
Below we list 8 possible interactions between electrons and positrons with their environment that may result in emission of some sort of radiation. For each interaction state the name of inter-action and type of radiation emitted followed by a brief description of the interaction.
(a)
Rapid deceleration of energetic electrons striking an absorber (target).
(b)
Direct interaction between high-energy electron and nucleus
(c)
Deceleration of electrons in a retarding potential in vacuum.
(d)
Deceleration of electrons in patients irradiated with photon or electron beams.
(e)
Acceleration of electrons in a linac waveguide.
(f)
Curved motion of electrons in circular accelerators.
(g)
Deceleration of positrons in positron emission tomography (PET).
(h)
Atomic polarization effects when electrons move through transparent dielectric absorber.
SOLUTION:
(a) Rapid deceleration of energetic electrons striking an absorber (target). As energetic electrons strike an absorber (target) they penetrate the target and may experience various Coulomb-type interactions with atoms of the target. These interactions are either elastic or inelastic collisions between the incident electrons with either orbital electrons of the target or nuclei of the target. Of the four types of Coulomb interactions, inelastic collisions between incident electron and orbital electrons result in the so-called characteristic (fluorescence) x rays and inelastic collisions between incident electron and nucleus of the target result in the so-called bremsstrahlung x rays. Energy of a characteristic x-ray photon is equal to the orbital energy transition that produced the photon and this energy is characteristic of the target atom, hence the name “characteristic radiation”. Energy of bremsstrahlung photon depends on the kinetic energy of the incident electron and the strength of the Coulomb interaction between the incident electron and the nucleus.
Thus, the x-ray spectrum produced by electrons striking a target has a continuous bremsstrahlung component ranging from 0 to kinetic energy of incident electrons and superimposed on this continuous spectrum are several discrete spectral lines characteristic of the target material.
The photon spectra used in medicine for diagnosis of disease typically range from 50 kVp to 150 kVp, while x-ray spectra used in radiotherapy for treatment of disease fall into three somewhat arbitrarily defined ranges: superficial x rays from 50 kVp to 80 kVp and orthovoltage x rays from 80 kVp to 350 kVp, both types generated by x-ray machines, as well as megavoltage x rays from 4 MV to 25 MV, generated mainly by linear accelerators and less commonly by microtrons and betatrons.
(b) Direct interaction between high-energy electron and nucleus. Many types of interaction are available to energetic electrons as they penetrate a target and interact elastically or in-elastically with orbital electrons and nuclei of the target atoms. Most of these interactions are of the Coulomb type and the inelastic interactions with orbital electrons are followed by characteristic x rays, while inelastic interactions with nuclei are accompanied by bremsstrahlung x rays, as discussed in (a).
In addition to these effects, the incident electrons may also undergo direct nuclear interaction with target nuclei and precipitate emission of neutron or proton through nuclear reactions labeled as: (e, n), (e, p), and (e, np) accompanied by transmutation of the target nucleus from stable to radioactive or from highly radioactive to less radioactive or even stable. Transition from stable to radioactive state is of concern in activation of linac components in high-energy radiotherapy, while transition from radioactive to more stable configuration is of great interest in the possible decontamination of highly radioactive waste.
The bremsstrahlung photons produced in electron–nucleus inelastic collision may also have a nuclear interaction with target nuclei of the type (γ,n), (γ,p), and (γ,np), similar to direct electron interactions with target nuclei of the type (e, n), (e, p), and (e, np), discussed above. These photon interactions have similar outcomes as the electron–nucleus interactions and are called photonuclear reaction or photodisintegration. In addition to these, photons may also trigger fission reactions in heavy nuclei and this type of interaction is referred to as the photofission reaction (γ,f). Thus, direct electron–nucleus interactions and reactions between electron-generated bremsstrahlung photon and nucleus have essentially identical outcomes; however, the cross sections for photonuclear reaction are significantly larger than those for associated direct electron–nucleus reactions.
(c) Deceleration of electrons in a retarding potential in vacuum. Deceleration of electrons in a retarding potential results in microwave radiation in contrast to deceleration of light charged particles in solid targets that produces bremsstrahlung x rays. Deceleration of electrons in a retarding potential is used in magnetrons and klystrons that serve as sources of radiofrequency power and amplifiers of radiofrequency power, respectively, for particle acceleration in linear accelerator (linac), microtron, and synchrotron.
(d) Deceleration of electrons released or produced in patients irradiated with photon beams. When high-energy photon beams are used in patient irradiation, photons interact with atoms of the irradiated tissue and, in these interactions, energetic electrons (in photoelectric effect, Compton effect, pair production) and positrons (in pair production) are set in motion in tissue. As the energetic electrons and positrons travel through tissue, they experience standard Coulomb interactions with tissue atoms and in some of these interactions bremsstrahlung photons are produced. These photons carry their energy out of the irradiated volume and contribute to the unwanted total body dose that the patient receives during the treatment of localized disease.
(e) Acceleration of electrons in a linac waveguide. The electron is injected into a linac waveguide with a typical kinetic energy of 25 keV that it receives in the electron gun. In the waveguide the electron is then accelerated from 25 keV up to the nominal energy of the linac which is of the order of several MeV or even larger in research linacs. According to Larmor relationship a charged particle accelerated or decelerated will lose part of its kinetic energy in the form of photons. Thus, at least in principle, an electron following a rectilinear motion in a linac waveguide should emit some bremsstrahlung radiation during its acceleration process. It turns out, however, that the emission of this unwanted radiation is minimal and is accounted for when the total leakage radiation produced by a clinical linac is measured.
(f) Motion of electrons in circular accelerators such as betatron, microtron, and synchrotron as well as storage ring implies curved motion of charged particles in transverse magnetic field resulting in circular paths and constant acceleration. According to Larmor relationship, this results in emission of radiation that is called synchrotron radiation or “magnetic bremsstrahlung” and is typically of lower energy than standard bremsstrahlung. In comparison with synchrotron radiation, the accelerations in production of standard bremsstrahlung are random and also much stronger.
On the one hand, in circular electron accelerators the synchrotron radiation is an unwanted result of the electron acceleration process in circular paths and, on the other hand, storage rings may be designed such that they generate synchrotron radiation for use in science and medicine.
(g) Deceleration of positrons (slowing down before annihilation) in positron emission tomography (PET) imaging studies of human organs results in unwanted stray radiation through the bremsstrahlung process between the positron and nuclei of tissue atoms. In a clinical PET test a positron-emitting radionuclide is administered to the patient by injection or inhalation. The radionuclide circulates through the bloodstream to reach a particular organ. The positrons emitted by the radionuclide have a relatively short range in tissue but most of them lose all of their kinetic energy either through collisions with orbital electrons of tissue atoms (collision loss) or nuclei of tissue atoms (radiation loss). The positron eventually annihilates with an orbital electron of a tissue atom and two annihilation quanta used for PET imaging are emitted.
(h) Atomic polarization effects when electrons move through a transparent dielectric absorber with a uniform velocity that exceeds the speed of light in the dielectric absorber result in visible light referred to as Čerenkov radiation. The efficiency for production of Čerenkov radiation is several orders of magnitude lower than the efficiency for bremsstrahlung production.
The emitted Čerenkov radiation does not come directly from the charged particle. Rather, the emission of Čerenkov radiation involves a large number of atoms of the transparent dielectric medium that become polarized by the fast charged particle moving with uniform velocity through the medium. The orbital electrons of the polarized atoms are accelerated by the fields of the charged particle and emit radiation coherently along the surface of a forward directed cone centered on the charged particle direction of motion.
14.4 Traditional Sources of X Rays
14.4.Q1 (292)
William Crookes is one of the prominent scientists of the 19th century, best known for his invention in the early 1870s of the “cathode ray” tube, now referred to as the Crookes tube. Crookes tubes were used in experimental physics for almost 50 years and are a precursor to modern vacuum tubes and cathode ray TV tubes.
(a)
Describe the basic principles of the Crookes tube.
(b)
Describe the difference between the Crookes tube and Crookes x-ray tube.
(c)
List and briefly describe at least 3 of the many seminal physics experiments that were carried out with Crookes tubes at the end of 19th century and the beginning of 20th century.
SOLUTION:
(a) Crookes tube is an electric discharge tube consisting of a sealed glass envelope that is evacuated to an air pressure between 0.005 Pa (4×10−5 torr) and 0.1 Pa (7.5×10−4 torr) and incorporates two electrodes (cathode and anode) connected to an external high voltage DC power supply. During the first three decades after the invention of the Crookes tube, many important experiments were carried out with the tube, and physicists soon established that the positively charged anode attracted unknown rays (referred to as “cathode rays”) originating in the cathode. Physicists were studying the cathode rays of Crookes tubes for many years but the understanding of their exact nature eluded them until Joseph J. Thomson in 1897 established that they were a new species of particle, negatively charged, and with mass of the order of 1800 times smaller than that of the hydrogen ion. Thomson called the new particle electron and succeeded in measuring the ratio between its charge and mass.
The basic principles of Crookes tube are now understood as follows: When high voltage is applied to the tube, electric discharge in the rarefied residual air inside the tube ionizes some air molecules. Positive ions move in the electric field toward the negatively charged cathode and create more ions through collisions with air molecules. As positive ions strike the negatively charged cold cathode, electrons are released from the cathode, move toward the anode in the electric field that is present between the cathode and the anode, and strike the anode. In contrast to a hot cathode that is based on thermionic electron emission, a cold cathode of a Crookes tube emits electrons upon impact by positive ions.
(b) Crookes x-ray tube. There is no essential difference in design between the Crookes tube and the Crookes x-ray tube. Both terms refer to the so-called “cathode ray” tube that Crookes developed in 1870s for investigation of electrical conductivity of gases at low pressure. However, after Röntgen’s discovery of x rays, it became apparent that a Crookes tube produced not only “cathode rays” but also a “new kind of ray” that became called x ray, so the description of the Crookes apparatus was expanded to encompass the “new kind of ray” and became known as Crookes x-ray tube.
(c) Of the many discoveries that originated through experimental work based on Crookes tube, three that seem the most important and were recognized with Nobel Prize in Physics are:
(1)
Discovery of x rays by Wilhelm K. Röntgen in 1895 (Nobel Prize in Physics—1901).
(2)
Discovery of electron by Joseph J. Thomson in 1897 (Nobel Prize in Physics—1906).
(3)
Oil-drop experiment and determination of electron mass by Robert A. Millikan in 1913 (Nobel Prize in Physics—1923).
(1) Discovery of x rays. In November 1895 Wilhelm K. Röntgen, a German physicist working at the University of Würzburg, discovered serendipitously that a Crookes tube, in addition to “cathode rays”, generated a new kind of ray which penetrated the tube housing and behaved in a very peculiar fashion outside the tube. For example, the rays were inducing fluorescence in platinocyanide crystals stored on a shelf across the laboratory. They were also capable of exposing photographic film and had the ability to penetrate opaque objects including hands, feet, and other parts of the human body. Röntgen named the unknown radiation x rays and soon thereafter the “new kind of rays” were introduced in medicine for diagnostic purposes. Röntgen’s discovery ushered in the era of modern physics and revolutionized medicine by spawning three new specialties: diagnostic imaging and radiotherapy as specialties of medicine as well as medical physics as a specialty of physics. For his discovery Röntgen received many honors and awards that culminated in his receiving the inaugural Nobel Prize in Physics in 1901.
The exact nature of x rays remained a mystery for a number of years until in 1912 Max von Laue, a German physicist, showed with a crystal diffraction experiment that x rays were electromagnetic radiation similar to visible light but of much smaller wavelength. Subsequently it became apparent that when the “cathode ray” electrons strike the anode (target), they undergo interactions with orbital electrons and nuclei of the target and some of these interactions result in characteristic and bremsstrahlung photons, respectively, that form the x-ray spectrum.
(2) Discovery of electron. In 1897 Joseph J. Thomson, a British physicist from the Cavendish Laboratory of Cambridge University, proposed that “cathode rays” observed in Crookes tubes were actually very small constituents of atoms and called them negatively charged particles or corpuscles. This was a very bold speculation at a time when atom was considered the smallest building block of matter and, as such, indivisible. Further experiments have shown that Thomson’s speculation was correct and the negatively charged corpuscles forming cathode rays were accepted as building blocks of all atoms and were called electron. However, Thomson’s hypothesis that electrons were the only building blocks of atoms was proven wrong with the subsequent discovery of proton and neutron as additional constituents of the atom.
Thomson could not measure directly the mass m e and charge e of the electron; however, he succeeded in determining their ratio, the specific charge of the electron e/m e, by measuring the deflection of electron trajectories in electric field
and magnetic field
both applied to a modified Crookes tube perpendicularly to the electron trajectory. Thomson’s experiment consisted of 3 stages:
The measured specific charge of the electron of e/m e≈1.7×1011 C/kg was several orders of magnitude larger than the then largest known specific charge of positive hydrogen ion (now known as proton) of
. The ratio of 1700 between the two specific charges could be explained either by much larger charge of the electron than hydrogen ion or by a much smaller mass of the electron compared to mass of the hydrogen ion. Thomson was convinced that the difference was caused by the much smaller mass of the electron compared to hydrogen ion, and it later turned out that his reasoning was correct. Thomson received the 1906 Nobel Prize in Physics “in recognition of the great merits of his theoretical and experimental investigations on the conduction of electricity by gases”. The currently accepted value for the specific charge of the electron is e/m e≈1.759×1011 C/kg, indicating that Thomson’s result of ∼1.7×1011 C/kg was quite reasonable if one considers the type of equipment in use for physics measurements more than a century ago.


(i)
First he proved that, at sufficiently low air pressure in the Crookes tube, the electric field
and magnetic field
affected the trajectory of electrons in the tube.


(ii)
Next, he balanced the electric force
and magnetic force
to achieve zero deflection of the electron trajectory with non-zero electric and magnetic fields. From F E=F B and the known
and
he determined the velocity υ of the electrons in the tube as
.





(iii)
Then, he turned off the magnetic field, measured the deflection of the electron trajectory as a result of electric field
, and from the measured deflection and known velocity of the electrons determined the specific charge of the electron e/m e as ∼1.7×1011 C/kg.

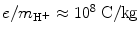
(3) Determination of electron charge e. In 1909 Robert A. Millikan, an American physicist, carried out at the University of Chicago his now-famous oil-drop experiment with which he determined the charge of the electron and proved experimentally the quantization of electric charge. Millikan’s experimental apparatus was simple and consisted of two chambers (upper and lower) connected with a small hole. An oil droplet atomizer was connected to the upper chamber and the hole allowed some small oil droplets to fall from the upper chamber into the lower chamber equipped with two electrodes connected to a variable DC power supply. The movement of oil droplets in the lower chamber was traced with a microscope used to establish the droplet speed and size.
Charge was applied to droplets randomly through irradiation of the air in the lower chamber using a Crookes x-ray tube. X rays ionized the air producing positive ions and electrons. Free electrons produced by ionizing radiation in air do not move freely in air, rather, they attach themselves to molecules of oxygen to form negative ions. The presence of oil droplets in ionized air also allowed free electrons to attach themselves to oil drops, changing some oil droplets from neutral to charged state.
Oil droplets were observed with the microscope and once a given oil droplet was identified the electrode potential was varied until the droplet stopped falling and was suspended in mid air. This indicated that the vertical electric force eE balanced perfectly the pull of gravity on the droplet, i.e., its weight mg. Droplets that did not acquire any electrons would not be affected by the electric field and would continue to drop; droplets that acquired more than one electron charge would require a lower electric potential to achieve suspension with no motion in air.
After numerous experiments Millikan determined that the electron charge e (elementary charge of electricity) was e=1.59×10−19 C which is within 1 % of the value e=1.602×10−19 C accepted today. He also noticed that there was variation in measured charge on oil droplets; however, the measured charge was always e=1.59×10−19 C or an integer multiple of this number. This means that electric charge in nature is quantized and its lowest value is e=1.59×10−19 C. Millikan received the Nobel Prize in Physics in 1923 “for his work on the elementary charge of electricity and on the photoelectric effect”.
14.4.Q2 (293)
A typical x-ray system used in science, medicine, or industry consists of five major components: (1) X-ray tube, (2) X-ray generator, (3) Control console, (4) Object under study, and (5) Image receptor.
(a)
Briefly describe the role of the five basic components of an x-ray system.
(b)
Draw a basic schematic diagram of an x-ray tube and briefly describe its major components.
(c)
Discuss the three x-ray tube designs that have been in use for x-ray production with x-ray tubes since Röntgen discovered x rays in 1895.
(d)
Prepare a table listing the following entries for the three x-ray tube designs: (1) X-ray tube type, (2) Cathode type (cold or hot cathode), (3) Electron ejection process from the cathode, (4) Air pressure in the x-ray tube, (5) Relative x-ray output, (6) Time of introduction and period of clinical use.
(e)
The anode of an x-ray tube is also called target. Briefly describe the main characteristics of a typical x-ray target.
SOLUTION:
(a) The basic components of an x-ray system are: (1) X-ray tube, (2) X-ray generator, (3) Control console, (4) Imaged object, and (5) Image receptor.
(1) X-ray tube serves as the source of x rays that are produced in the tube by relatively high energy electrons striking the tube anode, also called the x-ray target. The electrons are generated in the tube cathode and accelerated toward the target by the electrostatic field between the tube anode and the tube cathode. In the x-ray target, electrons suffer rapid deceleration and through interactions with target atoms produce x-ray photons: characteristic x-ray photons in electron–orbital electron interactions and bremsstrahlung x-ray photons in electron–nucleus interactions.
(2) X-ray generator (also referred to as high-voltage power supply) provides current at DC high voltage used for acceleration of electrons in the x-ray tube, thereby controlling the quantity and characteristics of x rays that the x-ray tube emits. X-ray equipment requires an adjustable high voltage ranging from ∼50 kV to ∼150 kV for standard x-ray procedures. Some special procedures are carried out with voltage below or above this range.
The DC high voltage in an x-ray machine is usually produced from a low voltage AC power grid by first increasing the AC voltage with a step-up transformer and then applying a rectifier circuit to obtain DC high voltage. Several methods are used for DC rectification and the objective of these is to deliver DC voltage with as small voltage variation (also called ripple) as possible. Typically, an increased sophistication in circuitry of an x-ray generator increases its cost but decreases its output voltage ripple and brings the output voltage closer to perfect DC potential. The high frequency x-ray generator currently represents the state-of-the-art choice in diagnostic x-ray systems for delivery of optimal DC potential.
(3) Control console is used to select the operating parameters of the x-ray generator, such as mode of operation, kilovoltage, tube current, and exposure time. These parameters in turn control the quantity and quality of the x-ray beam used for imaging of the object.
(4) Imaged object under study can be a patient or an inanimate object undergoing a diagnostic test intended to provide nondestructive testing of interior organs or structures. Thus, from a technical perspective the imaged object becomes part of the x-ray system. The acquisition of the desired information depends heavily on the characteristics of the imaged object and appropriate choice of parameters to be used in the imaging study. One should note that only a small fraction of energy expended to produce the x rays will be carried by the x rays, as most of the energy goes into heat that must be dissipated from the target. Furthermore, most of the energy carried by the x rays is actually absorbed by the imaged object and only a small fraction is transmitted through the object and carried to the image receptor to form the latent image.
(5) Image receptor is a series of devices that convert into visible image the radiographic information contained by the x-ray beam emanating from the imaged object. Various devices have been developed as image receptors during the more than 100 years of x-ray imaging:
In computed radiography the image receptor is the digital imaging cassette that stores the latent image on a special reusable plate coated with photostimulable phosphor (barium fluorohalide). For readout, the plate is transferred to a laser reader that scans the latent image with red light and renders it visible in blue light that is captured by a photodetector and converted to a digital signal for viewing.
(i)
The most common image receptor in the past during the era of analog conventional radiography has been radiographic film combined with special screens and a film developer. Upon exposure of the imaged object, film stored the latent image that was subsequently rendered visible through chemical development of the exposed film in a film developer.
(ii)
In the current era of digital imaging there are two options: computed radiography and digital radiography.
In digital radiography the latent x-ray image is captured with a flat panel detector containing a photoconductor that absorbs x rays in an amorphous selenium material and converts the latent image into digital signal.
(b) In Fig. 14.2 we show a schematic diagram of a simple x-ray tube with “cathode rays” (electrons) emanating from the cathode and accelerated toward the anode (target). The electrons strike the anode and lose some of their kinetic energy (typically only 1 % or less) in the form of x rays. The high voltage power supply establishes the DC potential between the cathode and the anode. The x-ray tube is evacuated, however, the residual pressure in the tube depends on the tube design.
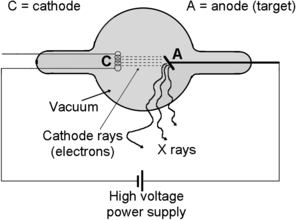
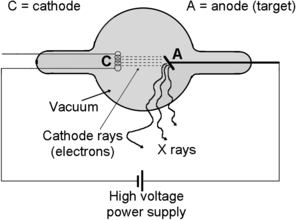
Fig. 14.2
Schematic diagram of a typical x-ray tube and its main components
(c) Three x-ray tube designs have been in use since 1895 when Röntgen discovered that cathode rays in Crookes cathode ray tube produce x rays in striking the tube anode. Basic components of x-ray tubes shown in Fig. 14.2 are the same for all x-ray tubes used to date; however, the three designs differ in their method for electron generation in the cathode, with two designs using a cold cathode and one a hot cathode. The three designs are: (1) Crookes x-ray tube—using cold cathode bombardment with positive air molecules to eject electrons from the cold cathode, (2) Coolidge x-ray tube—using thermionic emission of electrons from the hot cathode, and (3) Carbon nanotube (CNT) x-ray tube—using field emission for electron ejection from the cold cathode.
(1) Crookes x-ray tube is a Crookes cathode ray tube used for production of x rays. It is an electric discharge tube invented by British chemist/physicist William Crookes in the early 1870s. It consists of a sealed glass tube which is evacuated to an air pressure between 0.005 Pa and 0.1 Pa (4×10−5 tor and 7.5×10−4 tor) and incorporates two electrodes (cathode and anode) connected to an external DC power supply. When high voltage is applied to the tube, electric discharge in the rarefied air inside the tube ionizes some air molecules. Positive ions move in the electric field toward the cathode and create more ions through collisions with air molecules. As positive ions strike the cathode, electrons (cathode rays) are released from the cathode, move toward the anode in the electric field that is present between the cathode and the anode, and strike the anode. Investigating “cathode rays”, Röntgen in 1895 serendipitously discovered x rays as rays emanating from the anode bombarded with “cathode rays”.
For the first two decades after 1895, x-ray tubes used for clinical work were of the Crookes tube type; simple in design but suffering from severe practical problems related to the magnitude and reliability of the x-ray output. Low x-ray output combined with large fluctuations in x-ray output and difficulties in controlling the output were the main drawbacks of Crookes x-ray tubes.
(2) Coolidge x-ray tube. In 1913 William Coolidge, an American physicist, introduced a new x-ray tube design based on a hot cathode which significantly improved the reliability and performance of clinical x-ray tubes. Almost 100 years later, Coolidge’s hot cathode idea still provides the basis for design of modern x-ray tubes.
The hot cathode consists of a filament made of a high melting point metal, typically tungsten (melting point 3422 °C) or a tungsten based alloy, heated to a relatively high temperature to serve as source of electrons. The hot cathode emits electrons thermionically (see Sect. 1.27) in contrast to the cold cathode of the Crookes x-ray tube in which positive air ions striking the cathode trigger the generation of electrons. Another important difference between the Coolidge tube and the Crookes tube is that the Coolidge tube operates under high vacuum of the order of 10−4 Pa to prevent collisions between electrons and molecules of air and also to prevent filament deterioration because of oxidation.
The main advantages of the Coolidge x-ray tube are its stability and its design feature that allows the external control of the x-ray output. The hotter is the filament, the larger is the number of emitted electrons. The filament is heated with electric current; increasing the filament current increases the filament temperature and this in turn results in an increase in number of thermionically emitted electrons. This number of emitted electrons is proportional to the number of electrons accelerated toward the anode (tube current) and this in turn is proportional to the number of x rays produced in the anode (x-ray output). Increasing the high voltage potential between the anode and the cathode increases the kinetic energy of the electrons striking the target (anode) and this increases the energy of the emitted x rays.
(3) Carbon nanotube based x-ray tube. The Coolidge hot cathode improved significantly the x-ray tube performance, however, hot cathodes have some drawbacks, so that for decades, concurrently with improvements in hot cathode technology, search was on for alternative, more practical, and cheaper source of electrons preferably based on cold cathode design.
Field emission (see Sect. 1.28.2), which allows emission of electrons from the surface of a solid under the influence of a strong electric field, seems an excellent candidate for a practical and efficient cold cathode design. Attempts in this direction have been made for decades; however, the use of extremely small metal tips to achieve the large local electric field always resulted in electrodes that were unreliable, relatively inefficient, and not durable enough for routine x-ray tube operation.
During the past decade, a new generation of carbon based material called carbon nanotube (CNT) has been developed in nanotechnology laboratories and showed great promise for use as cold cathode-type electron source. Carbon nanotubes are ordered molecular structures formed by carbon, yet different from the two well-known carbon forms: graphite and diamond. They are molecular scale tubes with typical diameter of a few nanometers and a height of up to a few millimeters. The tubes have remarkable electronic properties and special physical characteristics that make them of great academic as well as potential commercial interest. They are extremely strong, yet flexible as well as light and thus hold promise for aerospace applications. Depending on their structure, they can behave like metal with conductivity higher than copper or like semiconductor potentially useful in design of nanoscale electronic devices. CNTs are mechanically, chemically, and thermally extremely robust and, since they also form atomically very sharp tips, they are also very efficient field emission materials for use as cold cathode electron source in x-ray tubes.
Miniature x-ray tubes using CNT cold cathode design are already commercially available. They generate electrons at room temperature and provide controllable as well as stable output currents and respectable life of the cathode. They can be used for “electronic brachytherapy” in medicine where they replace sealed radionuclide sources as well as in space exploration for performing remote mineralogical analyses on solid bodies of the solar system. Use of cold cathode for high power x-ray tubes in medicine and industry, however, if it happens, is far in the future, since the technology of CNT production is still in a rudimentary stage and field emission cold cathodes are currently no match for the standard Coolidge hot cathode x-ray tube design.
(d) Table 14.2 lists the main characteristics of the three main types of x-ray tube categorized according to cathode design: Crookes x-ray tube, Coolidge x-ray tube, and Carbon nanotube (CNT) x-ray tube. However, Crookes x-ray tubes are no longer is use and CNT x-ray tubes are not ready yet for mainstream use. This means that Crookes tubes are on the list because of their historical significance and CNT x-ray tubes are on the list because of their potential for practical use in the future. Currently, the vast majority of x-ray tubes used in medicine and industry are of Coolidge type design using a hot cathode.
Table 14.2
Main characteristics of x-ray tubes: Crookes x-ray tube, Coolidge x-ray tube, and carbon nanotube (CNT) x-ray tube
(1) | X-ray tube type | Crookes x-ray tube | Coolidge x-ray tube | CNT x-ray tube |
---|---|---|---|---|
(2) | Cathode type | COLD | HOT | COLD |
(3) | Electron ejection | Cathode bombardment with positive ions | Thermionic emission | Field emission in strong electric field |
(4) | Air pressure | Intermediate vacuum 0.005 Pa to 0.1 Pa | High vacuum ∼10−4 Pa | Intermediate vacuum ∼10−4 Pa |
(5) | Relative x-ray output | LOW and erratic; depends on air pressure inside tube | HIGH, variable and steady; depends on cathode temperature | LOW; depends on CNT design |
(6) | Clinical use period | 1895 to ∼1920 | 1913 to present | Relatively new design |
Table 14.2 lists the following entries: (1) X-ray tube type, (2) Cathode type (cold or hot), (3) Electron ejection process, (4) Air pressure in x-ray tube, (5) Relative x-ray output, and (6) Period of clinical use.
(e) Electrons generated by the cathode bombard the target (anode) of an x-ray tube and a minute fraction of the electron’s kinetic energy (typically 1 % or less) is transformed into x rays (characteristic radiation and bremsstrahlung) and the rest into heat. The anode thus has three functions in an x-ray tube: (1) to define the positive potential in the x-ray tube, (2) to produce x rays, and (3) to dissipate the heat.
The anode material must have a high melting point to be able to withstand the high operating temperature and a relatively high atomic number for adequate x-ray production. Most common target materials for x-ray tubes are tungsten (wolfram) with atomic number Z=74 and melting point of 3422 °C and molybdenum with Z=42 and melting point of 2617 °C. Tungsten is used in x-ray tubes operating above 50 kV and molybdenum in x-ray tubes below 50 kV.
The two most important practical attributes of x-ray targets for imaging or radiotherapy are:
(1)
Small focal spot (as close as possible to “point source”) to minimize beam penumbra. A smaller beam penumbra results in sharper image in x-ray imaging and in better dose distribution in radiotherapy.
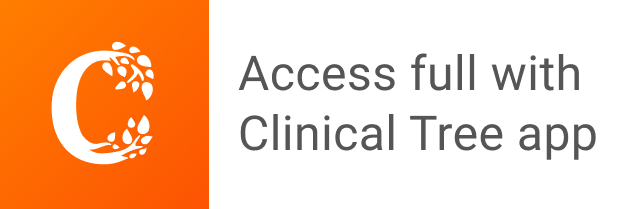