Introduction
Atherosclerosis of the peripheral arteries is recognized as a major cause of death and disability. It has been estimated that at least 8 million people in the United States have stenosis or occlusion of one or more of their lower limb arteries. Of this group, approximately 4 million are symptomatic, while a similar number of individuals have asymptomatic disease and are at future risk for compromised ambulation, arterial ulceration, and the need for revascularization. In addition, recent investigations suggest that 30% of smokers, diabetic patients over the age of 50, and those over 70 years of age suffer from some form of peripheral arterial disease. As such, it is important to define test procedures that are capable of detecting and localizing arterial disease, estimating the severity of arterial lesions, documenting critical ischemia, determining the potential for healing, and defining the therapeutic options.
Historically, arteriography has been the method of choice for the assessment of arterial disease affecting the lower limb and has served as the standard for planning reconstructive surgery or endovascular procedures. This invasive procedure has an associated, albeit low, morbidity and may underestimate or overestimate the functional significance of eccentric lesions. Arteriography has been supplanted in many facilities by three-dimensional magnetic resonance or computed tomographic angiography. Regardless of the procedure used, it must be recognized that none of these modalities is capable of accurately defining the functional impact of multilevel occlusive disease on tissue perfusion.
The noninvasive physiologic assessment of the lower extremity arteries was introduced in the 1960s as a valued mechanism for confirmation and localization of arterial disease, determination of disease severity, and definition of response to therapy. Recognizing that significant blockage in the limb arteries reduced blood pressure and blood flow to the tissues distal to the obstruction, investigators used a variety of diagnostic tools to document the physiologic alterations in pressure and flow. Although some of the earlier techniques are no longer used, indirect, physiologic testing remains the primary diagnostic method for assessing lower limb arterial disorders in the modern vascular laboratory. These nonimaging studies are complemented by duplex sonography that adds site-specific and quantitative diagnostic information.
Instrumentation
Directional, continuous-wave Doppler
Principles
Continuous-wave (CW) Doppler flowmeters can be used to detect the presence, quality, and direction of blood flow in the extremity arteries. As noted in Chapter 1 , the principle of Doppler ultrasound is based on the difference between the transmitted frequency and the frequency of the returning echoes (Doppler effect). Given the ultrasound frequencies that are used, the changes in frequency due to blood flow result in an audible frequency shift that is proportional to blood flow velocity. This relationship is expressed in the Doppler equation
Δf=2Vf0cosθc
As noted in earlier chapters, the ultrasound beam is more significantly attenuated with depth as frequency increases. This affects the selection of the transmitting frequency used for arterial interrogation. Given this, transmitting frequencies in the range of 5 to 12 MHz are most commonly used for evaluation of the lower extremity arterial system, with the lower-frequency CW transducers used for examination of deeper vessels such as the common femoral artery.
CW Doppler utilizes two crystals: one crystal continuously transmits sound waves, while the other continuously receives the returned signals from all moving targets within its path. These signals are summed and may be projected audibly or represented as an analog display. Care must be taken to avoid contamination of the arterial signal by superimposed signals from adjacent veins and to ensure that arterial phasicity and pulsatility are accurately represented by optimizing the transmit frequency, angle of insonation, gain, and analog display.
A normal, resting peripheral artery will demonstrate both forward and reverse flow components ( Fig. 12.1 ). This multiphasic flow pattern is most often displayed as an analog tracing. The graphic display represents the average of the frequency shifts that occur over time. Using a zero-crossing detector, blood flow direction can be displayed on a strip chart recorder and retained for later qualitative analysis. This device uses a frequency-to-voltage converter. The output of the voltage is proportional to the number of zero crossings. Every time the input signal crosses through zero in a positive direction, a tag is set. The tag is reset every time the signal crosses through zero in a negative direction. The Doppler frequency is estimated based on the number of times the tag is set every second. Several important deficiencies have been associated with zero-crossing detectors. Although the display is generally acceptable, it is very dependent on the signal-to-noise ratio, amplitude of the signal, and transient response. The information (mean frequency shifts) is not quantitative because the angle of insonation is not known. Because of this, low frequency shifts (low velocities) may be overestimated and high frequency shifts (high velocities) may be underestimated.
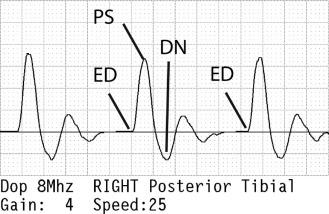
State-of-the-art CW Doppler flowmeters can detect blood flow velocities as low as 6 cm/s. CW Doppler flowmeters may fail to detect arterial signals in critically ischemic limbs with multisegmental occlusive disease. It may be difficult for the examiner audibly to differentiate low-velocity, minimally pulsatile, arterial flow signals in ischemic limbs from venous signals. In such cases, alternative methods that can evaluate tissue perfusion or tissue oxygen levels may need to be used in order to determine whether tissue perfusion is sufficient to promote tissue healing.
Although CW Doppler is recognized as a valued tool for assessing arterial blood flow, its technical limitations must be considered. Distorted waveforms may be recorded unless care is taken to optimize the position and angle of the probe with respect to the artery ( Fig. 12.2A and B ).
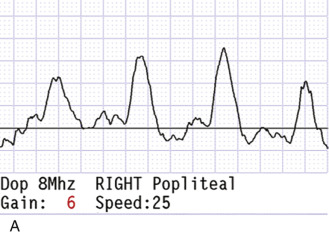
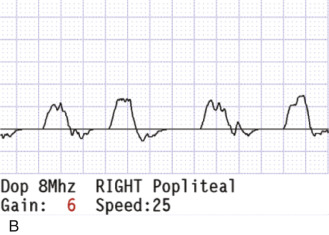
The signal may be attenuated by scar tissue or calcification of the arterial wall. Perhaps the most serious deficiency is the inability to control the sample volume depth (range ambiguity) in order to retrieve velocity information at a precise location within a designated vessel. This problem is overcome when pulsed Doppler is utilized for velocity assessment (see Chapter 15 ).
Continuous wave Doppler analysis
As shown in Fig. 12.1 , a normal resting peripheral arterial signal is multiphasic with one or more diastolic components. The initial systolic forward flow is followed by rapid deceleration to a brief period of early diastolic flow reversal, resulting from high peripheral vascular resistance and a negative pressure gradient. A second phase of forward blood flow during diastole is usually pronounced when peripheral resistance is decreased as a result of a downstream flow demand. Diastolic blood flow can be decreased or absent during this phase when there is increased distal resistance due to a distal lesion, vasoconstriction due to a low room temperature, or loss of compliance due to age-associated calcification and arterial wall stiffening.
The absence of early diastolic flow reversal suggests a forward flow demand ( Fig. 12.3 ). This is most often associated with vasodilation that occurs with flow-reducing arterial disease (>60% diameter-reducing lesions), but may also be seen with exercise-induced vasodilation or increased body temperature.
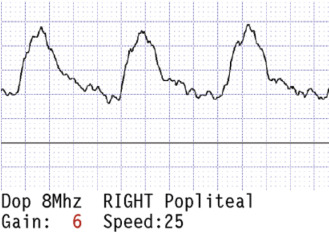
With intrinsic disease, arterial pressure is higher proximal to the site of narrowing and lower distally. The reduction in distal pressure is accompanied by a loss of kinetic energy distal to the stenosis. With disease progression, there is further loss of energy and pressure, which results in increased vasodilation. When flow-limiting stenosis is proximal to the site of Doppler interrogation, the waveform will be characterized by decreased amplitude and delayed systolic upstroke because of the increased time required for blood to bypass the stenosis through collateral channels. As severity of disease increases, vascular resistance continues to decrease distally and the waveform morphology will exhibit delayed diastolic runoff (bowing to the right). When lengthy occlusions or multilevel disease is present, the waveform becomes dampened with significant loss of amplitude ( Fig. 12.4 ).
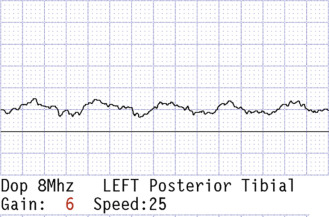
An arterial stenosis will alter the Doppler frequency shift and waveform morphology. Proximal to a severe stenosis, the waveform may appear normal if the lesion is well collateralized. When there are no collateral pathways, a “thump-like” signal with reduced amplitude, and little to no runoff, will be recorded ( Fig. 12.5 ).
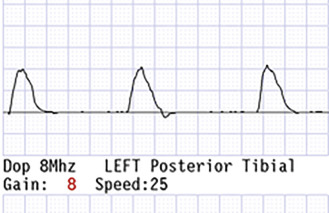
The Doppler signal obtained directly over a stenosis will demonstrate a high-frequency shift (velocity) at peak systole and loss of the early diastolic reverse flow component. A forward flow pattern will be present throughout systole and diastole. A high-pitched, harsh audible signal will be noted immediately distal to the stenosis. The poststenotic waveform is characterized by decreased frequency shift and forward diastolic flow. Multilevel disease may compromise blood flow so critically that the CW Doppler waveforms will be severely dampened or absent in cases of very decreased blood flow velocities below the detection threshold of CW Doppler.
Identification of flow-limiting disease and classification of disease severity may be enhanced by quantitative Doppler waveform analysis. Historically, several methods of waveform analysis have been used, including calculation of a pulsatility index (PI), inverse damping factor, pulse wave transit time, acceleration time (AT), and the Laplace transform analysis. Although each of these has met with varying levels of success and acceptance, only the PI and AT have retained popularity. The peak-to-peak PI (peak 1 frequency shift − peak 2 frequency shift / mean frequency shift) relates the peak-to-peak frequency shift (velocity) to the integrated mean frequency shift (velocity) and is independent of the angle of insonation. The relationship of peak-to-peak frequency shift and the integrated mean frequency shift is illustrated in Fig. 12.6 .
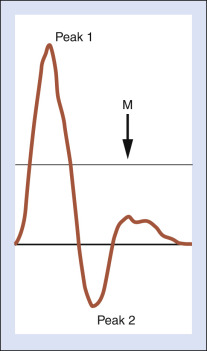
In the normal lower limb, the PI values increase from proximal to distal. The PI of the common femoral artery is normally greater than 5 and most often is between 6 and 7, whereas the popliteal artery has a PI between 7 and 9, and the posterior tibial artery PI range is 12 to 16. In the presence of a pressure-flow-reducing lesion, the reverse flow component of the Doppler waveform is absent (peak 2) and the PI decreases. Comparing their results to intra-arterial pressure measurements, Thiele and colleagues demonstrated that a common femoral artery PI greater than 4 was predictive of a normal aortoiliac segment. In the absence of superficial femoral artery occlusive disease, a common femoral artery PI less than 4 was highly predictive of flow-limiting aortoiliac disease.
Hemodynamically significant disease proximal to the common femoral artery can be identified by measurement of the systolic AT on the Doppler waveform ( Fig. 12.7 ). Normally, common femoral artery systolic rise time is rapid (<122 ms). Flow velocity is slower when blood must move around an area of blockage through high-resistance collateral pathways. In such cases, the systolic rise time is extended to greater than 144 ms. Van Tongeren and colleagues used the maximal systolic acceleration, ([peak systolic velocity – end-diastolic velocity] / AT) to identify successfully peripheral artery flow-limiting lesions in diabetic patients. A maximal systolic acceleration exceeding 10 m/s 2 excluded peripheral artery occlusive disease with a negative predictive value of 95%, whereas an acceleration of less than 6.5 m/s 2 had a positive predictive value of 99% for detecting flow-limiting disease. It is important to optimize the Doppler signal and angle of insonation because false-positive results are likely when the Doppler angle is greater than 60 to 70 degrees and the waveforms are dampened. The signal will also be attenuated and show both decreased AT and systolic acceleration in aortic stenosis.
- •
CW Doppler devices give only frequency shift information. Blood flow velocities are not assessed because the angle between blood flow and the ultrasound beam must be assumed.
- •
Waveform analysis is used to assess the state of arterial blood flow.
- •
Quality of the systolic upstroke indicates whether or not there is a significant proximal obstructive lesion.
- •
A rounded waveform shape and relative increase in diastolic signals indicate peripheral vasodilation due to significant inflow disease.
- •
Increased diastolic signals can also indicate increased demand due to exercise or inflammation.
- •
CW Doppler flowmeters may fail to detect arterial signals in critically ischemic limbs.
- •
The AT and PI can be measured on the waveform tracings and provide evidence of proximal arterial obstruction.
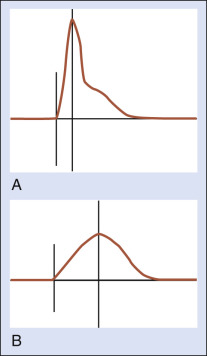
Plethysmography
Principles
Plethysmography records blood volume changes in tissues of the limbs or digits. Most commonly, the volume changes are related to normal alterations in the volume blood flow seen during the cardiac cycle or abnormal alterations secondary to pressure-flow-reducing lesions. Historically, a number of plethysmographic tools have been used to examine the lower extremity arterial and venous circulation. Although the majority of these are still in limited use, modern vascular laboratories most often use air-calibrated plethysmography (pulse volume recording [PVR]) and photoplethysmography (PPG).
Air-calibrated plethysmography
Changes in limb volume occur with passage of the arterial pulse pressure wave from the aorta into the arterial tree of the lower extremities. In systole, blood moves rapidly from the main arterial branches into the microcirculation, with a resultant increase in tissue perfusion and limb volume. During diastole, the pressure in the main arterial tree diminishes with subsequent reduction in limb volume. The cyclical changes in limb volume can be documented with PVR. This modality relies on the application of pneumatic cuffs at different levels of the lower extremity ( Fig. 12.8 ).
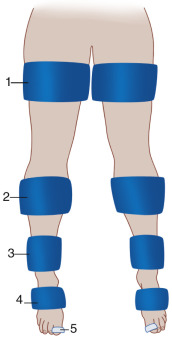
The cuffs serve as sensors for alterations in limb volume due to arterial inflow and venous outflow. The individual cuff is calibrated by inflating the cuff bladder to a pressure of approximating 65 mm Hg and with a volume sufficient to ensure full apposition of the cuff bladder to the skin.
During systole, cuff volume decreases and pressure increases as a consequence of the increased blood volume and pressure in the limb segment. In diastole, arterial inflow decreases and the pressure decreases as limb volume decreases and cuff volume increases. These pressure changes are recorded by a pressure transducer and translated into an analog recording that displays the amplitude and contour of the pulse wave. Although the frequency response of some devices approximates only 20 Hz, this has been shown to be sufficient for demonstration of the high-frequency components expressed in the arterial pulse pressure wave.
Pulse volume waveform analysis
The analog pulse volume wave contour parallels the intra-arterial pressure contour. The normal wave exhibits rapid systolic upstroke (anacrotic limb), a sharp systolic peak, a reflected wave (dicrotic notch) on the deceleration slope, and gradual runoff in diastole ( Fig. 12.9 ). The reflected wave signifies elevated peripheral resistance, which is expected in the normal resting muscular bed of the lower limb. Vascular resistance is reduced in response to any situation that elicits an increased flow demand (e.g., significant arterial stenosis or occlusion, exercise, or inflammation). As severity of disease progresses from single-segment 50% to 60% diameter-reducing stenosis to multilevel occlusive disease proximal to the recording cuff, the PVR waveform will exhibit a continuum of changes ( Fig. 12.10 ).
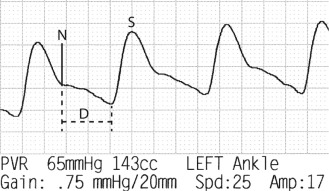
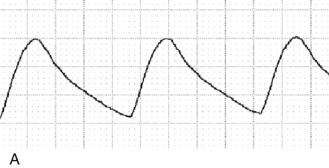
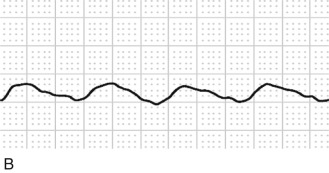
The amplitude of the pulse volume recording is dependent on the total flow and pulse pressure at a given limb segment. As such, the amplitude of the pulse volume wave provides clues to the presence of hemodynamically significant disease and the extent of compensatory flow in the microcirculation. Pulse volume amplitude is normally high in the absence of pressure-flow-reducing lesions. The amplitude is reduced when there is significant arterial obstruction proximal to the segment where the pulse wave is recorded. As disease severity increases, the amplitude of the waveform decreases. These findings reflect the associated reduction in pulse pressure and tissue perfusion. As such, whenever there is a significant change in the amplitude and contour of the PVR waveform compared with the more proximal recording, flow-limiting disease should be suspected. The offending lesion may be beneath the cuff or between the two cuff levels.
In situations where there is abundant flow in the tissue bed (e.g., well-developed collaterals, arterial-venous fistulas or malformations), the pulse volume waveform will demonstrate higher amplitude compared with the more proximal waveform recordings. For example, in the absence of flow-limiting disease in the superficial femoral artery, the amplitude of the PVR waveform recorded at the upper calf level will be higher than the amplitude of the thigh and ankle waveforms ( Fig. 12.11 ). This is the result of the greater volume occupied by arterial branches within the volume defined by the location of the PVR cuff, its size, and the transmitted pulse wave. Absence of the amplitude increase suggests superficial femoral artery occlusion. Distal superficial femoral artery obstruction should be suspected if the below-knee waveform demonstrates no increase in amplitude and the waveforms recorded at thigh level are normal. In addition, blood pressure, vasomotor tone, ventricular stroke volume, patient positioning, edema, and/or obesity can affect PVR waveform amplitude.
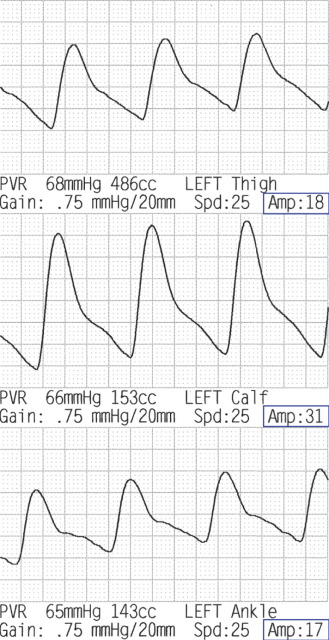
Recognizing the deficiencies associated with the subjective analysis of arterial plethysmographic waveforms, Scissons and his colleagues developed an objective analytical method and applied it to thigh PVR waveforms for identification of flow-reducing disease in the aortoiliac segment. Comparing their results with arteriography, these investigators achieved excellent sensitivity, specificity, and accuracy (85%, 93%, and 91%, respectively) for the identification of hemodynamically significant inflow arterial disease. They used thigh cuffs calibrated for appropriate pressure and volume, gain chosen for accurate waveform representation, and a strip chart speed of 25 mm/s. The thigh PVR waveforms were analyzed for acceleration time, maximum systolic amplitude deflection, downslope (deceleration) curvature, relative amplitude reduction, and gain compensation index. Acceleration time was measured from the onset of systole to mid-peak systole, recorded in seconds and then rounded to the nearest 0.04 s ( Fig. 12.12A ). The downslope curvature was based on the direction of curvature (inward, even, or outward) relative to a reference line drawn from mid-peak systole to end diastole (see Fig. 12.12B ). An inward curve or outward curve demonstrated a deflection equal to or greater than 50% in that direction relative to the baseline. An even curve exhibited an inward and/or outward deflection less than 50% relative to the reference line. The relative amplitude reduction (RAR) was determined from the ratio of the maximum peak systolic amplitude of the waveform minus the downslope waveform amplitude measured one-fifth of a second after the systolic mid-peak (see Fig. 12.12C ). This value was divided by the maximum peak systolic waveform amplitude and rounded to the nearest 0.04 s. The gain compensation index was obtained by dividing the maximum systolic waveform amplitude by the relative gain. Although a number of the parameters had predictive value for aortoiliac disease, an outward direction of downslope curvature was present in both diabetic and nondiabetic patients with inflow disease. This study indicates that objective analysis of plethysmographic waveforms may enhance diagnostic accuracy for detection of flow-limiting aortoiliac disease. The technique has not yet been widely investigated nor has it been applied to other segments of the lower extremity arterial tree. When validated, objective measurement of the plethysmographic waveform parameters could be adapted to computer software installed within commercial physiologic testing systems.
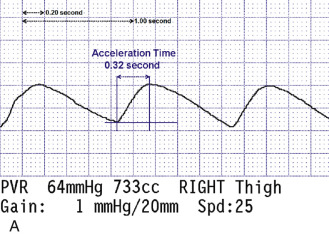
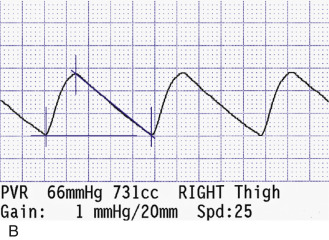
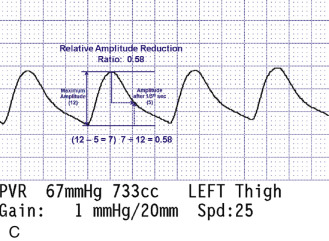
Photoplethysmography
Photoelectric plethysmography is used primarily for assessing the quantity of blood in the cutaneous circulation. This technology uses a light-emitting diode that transmits infrared light into the skin. A phototransistor detects the reflected signal from red blood cells coursing through the microcirculation in the tissues beneath the sensor. The changes in electrical resistance within the phototransistor are expressed as a waveform that displays signals throughout the cardiac cycle that are proportional to the number of red blood cells in the tissues. The PPG sensor is attached to the skin by using clear, double-stick tape or a tension-sensitive clip that houses the sensor. It is important to avoid attachment techniques that have potential for increased pressure on the sensor (clips that are applied over the sensor and Velcro straps or tape used to secure the sensor to the skin or digit) as this will force blood away from the tissue beneath the sensor. Although, in the truest sense of the term, PPG does not measure limb or digit volume changes due to alterations in blood flow, the pulse waveform morphology closely resembles the pulse volume waveforms recorded with PVRs ( Fig. 12.13 ).
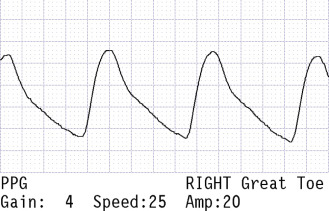
PPGs have shown value as sensors for arterial flow to facilitate measurement of digital pressures and for assessment of potential for healing wounds, ulcers, and amputation sites. The morphology of the plethysmographic waveform may be altered in situations that cause vasodilation or vasoconstriction such as temperature variations, inflammation, or certain medications such as vasoconstrictors or vasodilators. It is important to note that the PPG waveforms are not quantitative; they yield information about tissue perfusion that is based on subjective analysis of waveform morphology.
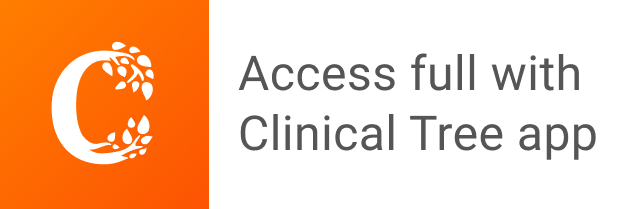