Fig. 6.1
Shift of 1 mm in prescription isodose line (green) in the anatomical, anterior right quadrant comparing ray-tracing plan (left) with Monte Carlo calculation (right)
The need for an algorithm that can accurately account for heterogeneities highly depends on the area of the body being treated. An additional factor to consider is the presence of inorganic materials from ancillary treatments. Examples are metal spine hardware or bone cement used to stabilize vertebral bodies.
6.6 Lung
The greatest heterogeneities occur with lesions in the lung. In the early clinical trials for lung cancer, algorithms for heterogeneity corrections were in their infancy; therefore, clinical trials such as RTOG-0236 required all internal tissues to be treated as though they possessed the same density as water. As the quality of dose calculation algorithms improved, the RTOG created a hybrid approach in which the treatment plan was based on the original homogeneous planning philosophy, while the actual delivered plan, based on a dose inhomogeneity calculation, was tracked. Currently, the clinical treatment planning practice varies from the traditional, homogeneous planning approach to hybrid models to the exclusive use of heterogeneity dose calculation algorithms.
The contrast in doses between the homogeneous planning approach and heterogeneity calculations can be substantial in lung. Wilcox et al. [12] have demonstrated a 20 % difference in maximum tumor dose between a pathway density correction algorithm and photon Monte Carlo [13, 14]. Davidson et al. [15] evaluated five treatment planning systems for the performance of their respective dose calculation algorithms in lung. The most widely used algorithm is the anisotropic analytic algorithm [16] (AAA). Increased processor speeds and better phase-space reduction methods have made it feasible to use photon Monte Carlo dose calculation algorithms clinically [13, 14]. A more recent development is the use of an algorithm belonging to the class of Linear Boltzman Equation Transport solvers. Early studies demonstrate accuracy comparable to AAA [17].
6.7 Other Materials
Treatment planning systems are currently not able to accurately calculate dose in the vicinity of metal, especially for smaller metallic structures such as stents and dental fillings. CT artifacts complicate the situation, although secondary reconstruction algorithms are being developed which, once implemented in the clinic, will significantly reduce the metal artifacts in simulation CT scans.
Strategies to minimize the effects of metal artifacts include density overwrites of the planning CT volume including the metal object and the imaging artifacts caused by it. The HU number is commonly set to water. For small implants such as aneurysm clips, stents, or dental fillings, a density overwrite is also recommended unless the planning system has been demonstrated to correctly compensate for the heterogeneity. In the evaluation of the plan, potential changes to the actual delivered dose compared to the dose displayed by the treatment planning system should be estimated and discussed based on published literature. In rare situations, e.g. with dental fillings, it is possible to evaluate the dose using in-vivo dosimeters and making adjustments to the treatment plan for subsequent SBRT fractions.
AVMs are most common in the brain, but can also occur, albeit rarely, in the spine. Commonly used therapeutic approaches are endovascular embolization with coils, glue or particles [18]. These treatments introduce high electron density material into the AVM. If the patient is then treated with SBRT for a recurrence, the difference in densities has to be considered during treatment planning [19].
Ethiodol, which is radiopaque poppy seed oil, is used as a contrast agent in TACE (Transarterial Chemoembolization) for liver tumors. As with embolization materials for AVMs, the higher electron density of these materials need to be considered in treatment planning.
Metallic stents are commonly used in the esophagus, pancreas and liver. Experimental measurements of dose in the vicinity of esophageal stents [20] have shown patterns of hot and cold spots depending on the construction material of the stent. Dose in the vicinity of an platinum aneurysm clip used in the brain has shown to be elevated by 10–20 %, while no changes in dose could be measured for other metals [21].
6.8 Plan Evaluation
Plans are evaluated based on whether the dose constraint goals are met. Some clinical case presentations do not allow for satisfying all goals simultaneously; therefore compromises have to be made on an individual patient assessment. To account for this variability, the RTOG has defined the concept of “minor” and “major” protocol violations, which the author highly recommends to adopt into clinical treatment planning for SBRT. For example, a planning target goal may be to limit the conformality index (CI) to < 1.2. A minor violation, which would still allow the patient to be included in the protocol, would be a conformality index 1.2 < CI < 1.4, while a CI >1.5 would prevent a patient from being included in the protocol or render the plan unacceptable.
6.8.1 Skin Dose
Acute skin toxicity has been reported for early-stage NSCLC SBRT treatments [3]. The authors reported that “Distance from the tumor to the skin on the patient’s back <5 cm (p = 0.006), treatment with three beams (p = 0.0007), and a maximum back skin dose >50 % of prescribed dose on the planning scan (p = 0.02) were all significantly associated with those patients that developed Grade 2 or higher skin reaction.” It is also important to carefully assess dose in skin folds, especially posterior skin folds which may not be as immediately obvious as anterior skin folds. For beams passing through the treatment couch and any setup devices, it is important to verify these to be accurately modeled by the treatment planning systems. Several couch models have been shown to change the dose delivered by oblique posterior beams by up to 6 %. Carbon fiber couches used in conjunction with electromagnetic tracking systems have been measured to be at the upper limit, or even exceeding, this level of dosimetric impact. The upcoming AAPM Task Group 176 “Dosimetric Effect of Immobilization Devices” will summarize a clinical review of the dosimetric effect of setup devices, including recommendations for clinical dosimetry.
6.8.2 Peripheral Dose
While the main focus of plan evaluation is naturally on the high-dose regions, the distribution of peripheral dose has significant impact both on short-term as well as long-term complication rates.
Many delivery devices, such as Gamma Knife, CyberKnife and Tomotherapy, are limited to one beam energy. When planning highly modulated beams from many directions, and especially when using non-coplanar or non-isocentric planning techniques, the planner must pay close attention to dose delivered in the periphery of the targeted treatment area. These “hot spots” can be more pronounced for obese patients, where the path-length from skin to target exceeds ~15 cm. In planning systems which use a calculation grid to define the body volume in which the dose is calculated and displayed for planning purposes, it is important for the planner to open up the calculation grid and evaluate the plan for peripheral hot spots. Effective ways to minimize the potential for peripheral hot spots include the use of a higher number of beams, limiting the number of MUs per beam direction, and using soft tissue constraints or phantom structures to control the peripheral dose.
Another consideration must be the peripheral dose distribution into peripheral OARs. With increasing cancer survival rates, considering low-dose regions to radiation sensitive organs may affect secondary cancer rates in the decades to follow. One of many examples of increased secondary malignancy caused by low peripheral doses to an OAR was published by Kleinerman et al. [22]. There are four sources for this dose: (1) Low-dose regions of the treatment plan itself, (2) Imaging dose, (3) Leakage, and (4) internal scatter. Leakage and internal scatter are machine-dependent. Imaging dose management is part of simulation and treatment delivery design. During the planning process, low-dose regions is the only peripheral dose parameter which can be influences by the planner under physician guidance.
6.9 Plan Standardization and Evaluation
The goal of designing optimum tumor coverage with the best organ sparing is a multi-criteria optimization problem. Until very recently, treatment planning systems (TPS) relied on a combination of planner skill and hardcoded optimization algorithm to try and find the best possible solution for each patient. Plan evaluation relied on physician and planner experience of what might be possible clinically; there was no scientific method to assess plan quality. More recently, improvements in informatics, specifically class-based database analysis and automation, have opened the door toward more quantitative plan quality analysis methods. In turn, these methods can be used as a tool to aid treatment planners in the analysis of plan quality and increase planning efficiency. For example, Moore et al. [23, 24] developed an experimental fit on the achievable lower limit for mean OAR dose based on OAR/PTV overlap. By making the lower OAR dose limit prediction available to planners, successive treatment plans showed lower variability and smaller deviation from the predicted minimum possible OAR dose without compromising PTV coverage.
Interactive multi-criteria IMRT planning [25], made possible by increased computing power and faster optimization algorithms, integrates plan optimization even earlier in the treatment planning process. Instead of calculating one or a few plans which are then selected and optimized, the treatment planning system will create a “plan bundle” based on the planner selected parameter space, i.e. lower and upper limits of dose constraints for all OARs. From amongst this plan bundle, the planner can then further restrict the Pareto surface by restricting multiple criteria through a user interface. Real-time update of the dose distribution provides the planner with an efficient method to assess and modulate DVH parameter restrictions for all structures involved in the treatment plan.
Another method for treatment planning standardization pulls treatment planning parameters such as beam angle, energy, DVH limits etc. from the existing library of clinically accepted plans to automatically create input parameters for a new treatment plan. This method allow the treatment planner to more efficiently set up the baseline treatment parameters from which to further optimize the treatment plan quality.
While these plan standardization and evaluation methods are in the early stages of clinical implementation, current treatment planning software development is rapidly moving towards integrating these tools into standard clinical practice. Minimizing the variability of treatment plans for the same treatment target is expected to lead to better treatment quality for patients by minimizing the influence of human factors on plan quality variance.
6.10 Tumor Dose and OAR Constraints
SBRT is a relatively new treatment concept, which means dose-fractionation schemes are still evolving. Biological effects studies are slowly becoming available as clinical trial results provide more information about complication rates. RTOG study protocols, AAPM TG-101 [26], QUANTEC [27–30] and an increasing number of peer-reviewed papers are available as clinical guidance to develop dose-fractionation schemes for SRS and SBRT.
In general, dose fractionation schemes for SBRT are adapted based on the position of the tumor relative to OARs. For targets which are isolated deep within a larger organ far away from other OARs, e.g. deep-seated liver lesions or peripheral lung lesions, maximizing the dose gradient to the healthy tissue of the affected organ becomes the planning priority. For targets abutting OARs, e.g. medial lung lesions or most pancreatic tumors, the OAR becomes the dose-limiting constraint in the treatment planning process.
6.10.1 Brain
Dose prescriptions schemes for brain tumors are based on several decades of experience with the Gamma Knife. Dose prescriptions are based on published complication rates based on lesion diameter [31]. The largest lesion diameter considered for SRS is typically 3 cm.
The development of frameless SRS has enabled physicians to develop fractionated treatment courses to alleviate the risk of necrosis associated with high doses in the brain. A special consideration to make when planning SRS to the brain is the fact that many cases are metastatic and therefore have a high probability of returning for further treatment, either to the same lesion or to new lesions. It is therefore advantageous to plan each case with consideration of dose to critical structures such as the lenses of the eyes, the optic chiasm and the brain stem regardless of their proximity, as well as to “normal” brain tissue.
6.10.2 Spine
The utilization of SRS for spine has increased with the implementation of frameless, image-guided SRS techniques [32]. The predominant fractionation scheme is still a single fraction of 10–18 Gy [33–36] although radiobiological considerations of cord tolerance for tumors in close proximity to the spinal cord or invading into the epidural space have led to fractionated treatments of 10 Gy × 3 or 80 Gy × 3. The tumor contour is typically standardized to include the complete vertebral body and the peduncles. The most significant OAR is of course the spinal cord. Contouring, and in consequence DVH constraints, vary between contouring the spinal canal (thecal sac) or the visible cord. Several authors have published retrospective studies on spinal cord tolerance [33, 37–39]; Choi et al. [40] published a study on cord tolerance for SRS in recurrent spinal malignancies.
The Canadian Association of Radiation Oncology (CARO) Stereotactic Body Radiotherapy task force has published guidance recommendations [41] for spine SRS which are listed in Table 6.1.
Table 6.1
CARO recommendations for SRS
Previously irradiated spine mets | 35 Gy/5 Fx |
Spine mets with no prior radiation | 30 Gy/4 fx |
Post-op patients (±prior radiation) | 24–26 Gy/3 Fx |
Selected primary spine tumors | 24–26 Gy/2 Fx |
No more than three consecutive vertebrae | 16–24 Gy/1 Fx |
6.10.3 Lung
The most widely used dose-fractionation scheme of 60 Gy in 3 fractions is based on the RTOG-0236 and RTOG-0618 protocols. These protocols used dose calculation algorithms based on homogeneous tissue models and have been shown to overestimate the dose by approximately 10 % [42]. As a result of these studies, some physicians have advocated using 54 Gy × 3 if inhomogeneity-correcting dose calculation algorithms are used. Other publications have studied outcomes as a function of tumor size, concluding a volume-adaptive dosing for lung SBRT could improve local control [43]. Table 6.2 summarizes selected society and clinical trial recommendations for lung SBRT dose-fractionation.
Table 6.2
Dose-fractionation recommendation from selected society recommendations and clinical trials
Target | Source | Dose Rx | |
---|---|---|---|
Lung | Medically inoperable T1/T2 N0M0 non-small cell | CARO | 60 Gy/8 Fx |
Lung mets | 50 Gy/5 Fx | ||
Tumors <5 cm | 48 Gy/4 Fx | ||
54–60 Gy/3 Fx | |||
34 Gy/1 Fx | |||
Inoperable stage I/II NSCLC, peripheral lesions | RTOG-0236 (phase II) | 60 Gy/3 Fx over 1.5–2 weeks | |
RTOG-0618 (phase II) | |||
Inoperable stage I/II NSCLC, peripheral lesions | RTOG-0915 (phase II) | 34 Gy/1 FX | |
Or | |||
48 Gy/4 Fx (consecutive days) |
Pneumonitis is the predominant reported toxicity for lung treatments. Because lung cancer patients are often elderly and have limited lung function, a V20 dose limit of 20 Gy is often used as a hard OAR constraint. Research efforts are underway to distinguish high-functioning from low-functioning lung regions with the goal to steer the OAR dose towards the low-functioning lung regions [44].
An added consideration is the dose delivered to the chest wall. Woody et al. [45] developed a predictive model for different dose-fractionation schemes based on a retrospective data analysis of 102 SBRT patients using a modified EUD model.
6.10.4 Pancreas
SBRT for Pancreas is warranted for resections, locally advanced cases, local recurrences and Oligometastatic Pancreas Cancers. The doses are designed by applying a tolerance-based approach, meaning that the proximity of the lesion within the Pancreases to critical structures, i.e., the stomach and duodenum, governs the total dose (Fig. 6.2) [46].


Fig. 6.2
The stomach and duodenum are the contiguous dose-limiting structures and the location of the lesion within the Pancreas dictates which dose scheme to assign (From Mahadevan et al. [46])
The liver, kidneys, spinal cord and bowel are among the other dose constraints to consider when planning SBRT to the pancreas.
6.10.5 Liver
SBRT for liver cancer is one of many possible therapeutic options to treat liver malignancies. Therefore, it is not utilized as often as e.g. SBRT for lung lesions. Early dose escalation studies [47–49] confirmed the safety of liver SBRT as non-surgical treatment option. Table 6.3 lists a selection of current society recommendations and clinical trials related to liver SBRT.
Table 6.3
Selected dose/fractionation recommendations for liver based on clinical trials and society recommendations
Hepatocellular CA <8 cm | CARO | 42–60 Gy/5 Fx |
Liver mets <6 cm and/or ≤5 lesions | 50 Gy/5 Fx | |
48 Gy/5 fx | ||
45 Gy/3 Fx | ||
Hepatocellular CA | RTOG-1112 (phase III) | 27.5–50 Gy/5 Fx, 24–72 h between Fx |
6.10.6 Prostate
Prostate SBRT is the most recent development in SBRT targets. The biological characteristics of early-stage prostate cancer require long-term follow-up studies to provide data on local control, late effects, and biochemically disease-free intervals. Because prostate SBRT trails at this point have only relatively short clinical follow-up, it is highly recommended limit the clinical use of this technique on a clinical trial basis. Until results are published, caution is advised in applying dose constraints for prostate SBRT.
There are currently two trials on hypo-fractionated prostate treatments. The major difference between them is that one aims for dose distributions with heterogeneities modeling prostate brachytherapy [50], while the other chooses a homogeneous dose approach [51]. The latter published their early prospective Phase II study results [52] which includes the treatment planning constraints found in Table 6.4.
Table 6.4
Typical dose fractionation and dose constraints for early stage low risk prostate cancer
Prostate | 7.25 Gy × 5, 3 × per week |
95 % coverage | |
5 mm margin (3 mm posterior) | |
10 % dose heterogeneity | |
Rectum | V50 < 50 % |
V20 < 80 % | |
V10 < 90 % | |
V5 < 100 % |
6.11 Planning in the Presence of Respiratory Motion and Deformation
Three common sites treated with SBRT are lung, liver and pancreas. In all three cases, respiratory motion can be significant. Larger tumors in the lung and liver also undergo deformation throughout the respiratory cycle. Accounting for respiratory motion and deformation in treatment planning strongly depends on the availability of 4D imaging studies, the respiratory motion compensation method chosen for treatment delivery, and individual patient considerations.
6.11.1 Imaging and Image Fusion for Planning
4D CT in planning is most commonly used to determine a gating window, and create an ITV (Internal Target Volume) based on the width of the gating window chosen for a particular patient. If compression is used to manage respiratory motion, the 4D CT can be used to generate a MIP image which determines the residual motion to be covered by the ITV.
If the treatment delivery system is capable of tumor and/or fiducial tracking instead of compression, breath-hold or gating, there are several additional issues to assess in the planning phase: deformation, rotation, and the relative position of the tumor to the OARs. Deformation and rotation margins can be determined by fusing the tumor center of mass in different respiratory phases and determining the ITV. The relative position of tumor to critical organs is much more difficult to assess with current technology. Deformable image registration algorithms have very limited suitability for this task, because relative voxel motion is not based on anatomically correct modeling of tissue motion.
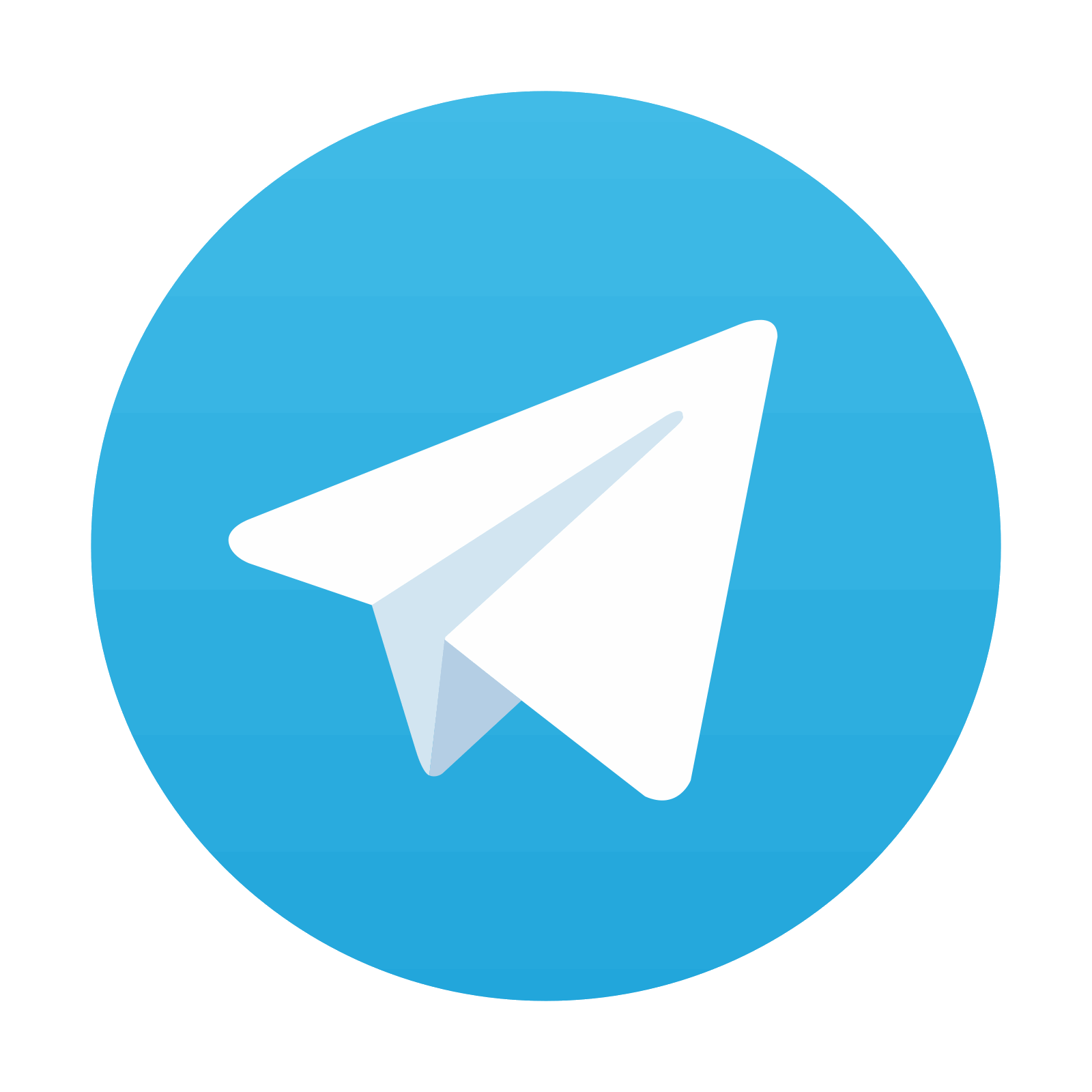
Stay updated, free articles. Join our Telegram channel
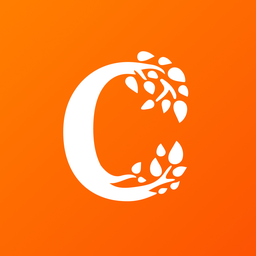
Full access? Get Clinical Tree
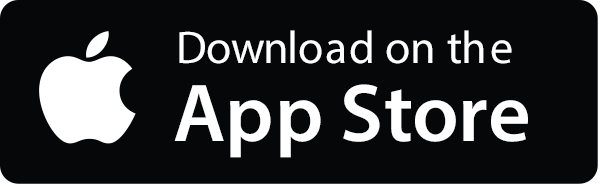
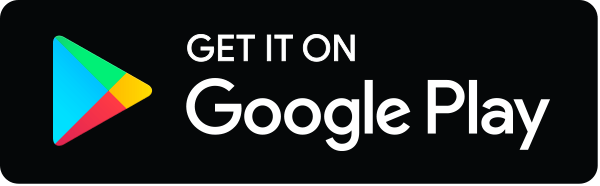